Fossil Fuels, the Building Industry, and Human Health
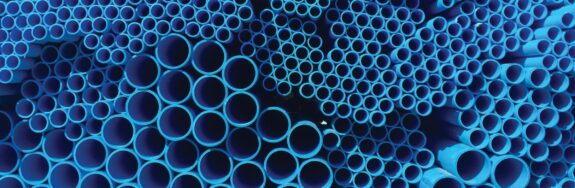
Nearly everything in our built environment is permeated by chemicals derived from fossil fuels. Yet there is little data about the health risks. This digest builds a case for more research, disclosure, and education.
The ubiquitous use of plastics in architectural design and construction obfuscates the very real human health risks which exist when polymers—derived from petroleum, coal, or natural gas—are used in the building industry. For more than fifty years, a majority of construction materials have been engineered using polymers for the purposes of achieving a range of advanced performance capacities. Even wood, the most traditional of materials, is widely manipulated using cold-cured synthetic resin glues for increasing its structural strength and moisture resistance. More typically, polyvinyl chlorides are used in plumbing supplies, exterior sheathing, interior surfaces, furniture, and landscaping.
Indeed, nearly everything in our built environment is permeated by chemicals derived from fossil fuels. And yet, more than half a century later, very little data is disclosed about the potential health risks associated with adopting such large quantities of nonrenewable and nonrecyclable plastics. Architects, engineers, builders, clients, and the general public are poorly informed on the toxic accumulation of synthetic polymers that are used in the building industry and pervade in our air, water, and physical bodies. In response, this digest identifies a set of policy priorities for the Architecture, Engineering and Construction (AEC) industry aimed at evaluating the Human Health (HH) impacts of using fossil fuel (FF) based polymers in the built environment. Central to this goal, is the creation of an industry based, Life Cycle Index of Human Health in Building (LCI-HHB).
Why Plastics
Why are plastics so ubiquitous? Why are they everywhere? Maybe because their properties are easily optimized to match an almost infinite variety of end use performance criteria. Polymer based wall finishes, floors, sealants, pipes, paints, flashing, and furniture are all derived from fossil fuel feedstocks that are chemically manipulated to become less or more transparent, porous, rigid, ductile, hypoallergenic, hydrophobic, or resistant to heat transfer.
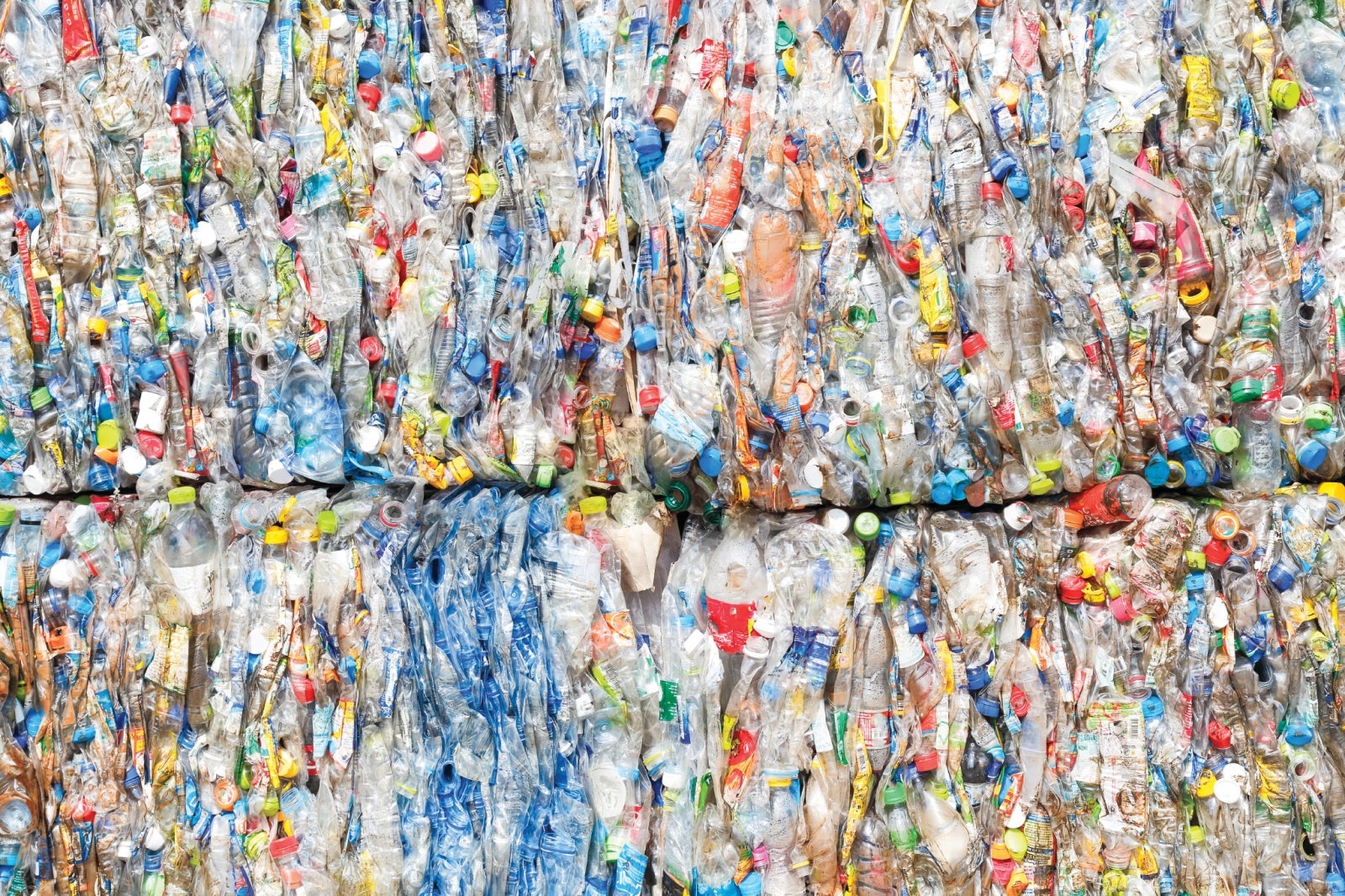
However, from a policy perspective, it is revealing that beyond the lab these highly engineered materials are typically referred to as simply, plastics, which is less a denotation of what they are than a description of their most common mechanical property: their ability to deform without breaking. Their larger material history, chemical definition, and associated health risks are so concealed to the layman that the following facts are poorly known; plastics are, by far, the most toxic of building materials, and the building material with the second greatest amount of embodied energy (Plastics=95 MJ/KG, Aluminum=170 MJ/KG) (Milne, 2013).
They are also complex chemical subjects. The vast majority of materials referred to as plastics are organic polymers: materials comprised of long chains of repeating molecular units, which—while incredibly tough—are able to glide past each other contributing to the material’s capacity for deformation.
Much of the versatility of plastics results from our ability to easily engineer interactions between these chains. The modifier organic specifies that these chains are linked by carbon atoms. In some cases, such as with polyethylenes, the linking unit is simply one carbon atom.
This organic distinction is important, as some polymers have repeating units of elements other than carbon, such as polysiloxanes (silicones). As this study is concerned with the link between fossil fuels and the building industry, we shall limit our definition of plastics to organic polymers.
Life Cycle of Carbon-based Plastics
Plastics have surprisingly carbon-intense life-cycles. The overwhelming majority of plastic resins come from petroleum, which requires extraction and distillation. Thereafter, the resins are formed into products and transported to market. All of these processes emit greenhouse gases, either directly or via the energy required to complete them. And the carbon footprint of plastics continues to accrue even after we’ve disposed of them. Dumping, incinerating, recycling, and composting (for certain plastics) all release carbon dioxide. All told, the emissions from plastics in 2015 were equivalent to nearly 1.8 billion metric tons of CO2 (Tasoff 2019).
According to the Center for International Environmental Law (CIEL), nearly “all plastic produced today (more than 99 percent) is manufactured from fossil fuel feed stocks,” including natural gas liquids and by-products from crude oil refining (CIEL 2019). Nearly 83% of all plastics produced by weight globally are sourced from just two industrial chemicals derived from fossil fuels: ethylene and propylene (CIEL 2019).
This industry sector will account for twenty percent of global fossil fuel consumption by 2050, which is why the largest oil and gas companies are also in the business of supplying the raw materials for plastics (CIEL 2019). As a result, the vast majority of building based plastics and polymer composites found in the environment today are chemically synthesized—from their base ingredients to their installed products—with the construction industry being the second largest consumer of plastics in the United States, consuming more than 12,000 million pounds per year.
In 2015, plastics contributed 1.8 billion metric tons of CO2, while in 2004 the entire building industry contributed but 2.236 billion metric tons of CO2 (Tasoff 2019, USGBC). And, as already noted, plastics are high in embodied energy. Given these numbers, building professionals who participate in the petrochemical to plastics cycle must be better informed of associated risks.
Undoubtedly, the industry is increasingly aware of the energy embodied in materials and the carbon emitted in their production. The applied science of Life-Cycle Assessment (LCA) or Life-Cycle Energy Assessment (LCEA) quantifies both embodied and operational energy of a product by studying the supply chain of all its source materials, as well as the product’s intended use and disposal (Bayer, 2010; Kohler 2013; Simonen 2014; Menzies 2007).
The building industry has embraced this expanded approach to material selection by incorporating an important role for LCAs in sustainability certification programs such as the Leadership in Energy and Environmental Design (LEED-USGBC), and rating systems such as the Living Building Challenge (International Living Future Institute) and Building for Environmental and Economic Sustainability (BEES).
And yes, traditional life-cycle assessments can sometimes result in the promotion of plastic in service to long term energy savings. For example, the total energy required to produce a PVC (polyvinyl chloride) window frame is about half that required for an aluminum alternative (Franklin Associates 1991); and expanded polystyrene (EPS) insulation is said to return—over a fifty-year period—200 times in energy savings compared to the energy consumed in its production (American Chemical Council 2009).
However, of all building materials, plastics have the most complicated life-cycle profile, involving embodied energy, operating energy, and elevated human health risks. And while advocating for the rejection of all plastic materials in the building industry solely due to their petrochemical origins is just as reductive as categorically championing their use because in some cases they favor operational energy savings. A method for judiciously evaluating possible energy savings against the risks posed to human health is still needed. To achieve this, an integrated life-cycle index for measuring the risk to human health posed by plastics is here advanced.
Existing LCAs and LCAEs poorly account for the very real costs to life and safety when sourcing, manufacturing, selecting, installing, using, maintaining, and disposing of plastic (Zheng and Suh 2019). Even Tally(™), the readily available LCA software supported by the U.S. Green Building Council, fails to acknowledge material toxicity amongst its impact categories. And while the BEES software developed by NIST (National Institute of Standards and Technology) integrates categories such as Ecological Toxicity, Indoor Air Quality, and Human Health data is only available for 230 material products associated with twenty-three building components.
A more holistic and transparent metric for itemizing, calculating, reporting, and evaluating the human health impacts of using polymerized materials sourced from fossil fuels is needed. Building-based plastics may be less expensive to produce and install, require less short-term maintenance, and have better water and thermal performance than comparable materials (metals, wood, and glass). Yet, they are nearly impossible to recycle, overrun our landfills, high in CO2 emissions, and in many cases toxic.
The last variable has no comparable and useful metric. Promoting the benefits of reduced operational energy is of little help to anyone not healthy enough to enjoy them. Needed is a comprehensive metric that includes human health risks of building with plastics, especially plastics as ubiquitous as polyvinyl chloride (PVC) (PlasticsEurope 2017).
Human Health Risks of Polyvinyl Chloride, an Example
Surely, polyvinyl chloride (PVC) is an incredibly versatile material used in roof scuppers, floor tiles, water pipes, wall paper, windows, and exterior cladding. Its two most important chemical feedstocks are ethylene and chlorine, sourced from petrochemicals and saltwater respectively (Perkins & Will 2019).
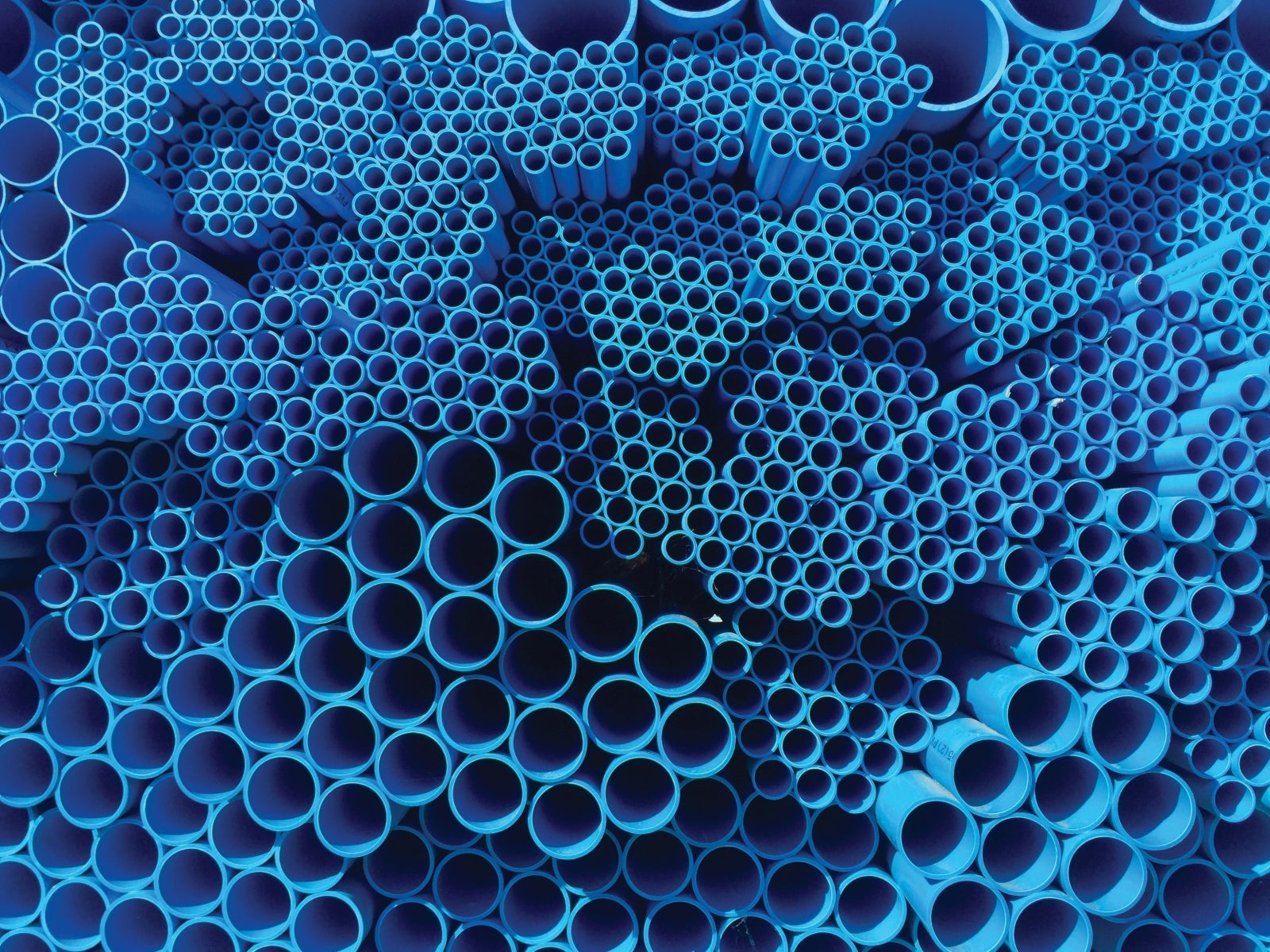
PVC production, as with many other synthetic fabrication processes, involves precursors that pose risks to human and environmental health. PVC’s monomer, VCM (vinyl chloride monomer) is a known carcinogen and must be manipulated in closed systems to protect workers from exposure (DOW 2019).
Those who work in manufacturing PVC products are protected from monomer precursors through regulation and industry practices (OSHA 2018); however, PVC is often plasticized (made less rigid) with chemical additives that can later leach into the environment (Crompton 2007, Lithner 2012). It is during the addition of phthalate plasticizers that are “loosely” connected to the PVC molecules that most of the toxicity we experience is introduced.
The vinyl industry has been under pressure to phase out the use of phthalate plasticizers, which are proven endocrine disruptors—a class of toxins that pose risks to reproductive health, immune response, and embryonic development (NIEH 2010). And, children are at the highest risk of asthma, rhinitis, and eczema when exposed to phthalates in their environment as dust, PVC contact surfaces, and flooring (Vesterberg 2005).
In addition, most PVC products are substantially non-recyclable; at end of life, they are typically incinerated or disposed of in landfills (Geyer 2017). Even in Europe, where recycling is highly championed, only three percent of post-consumer PVC is diverted from the waste stream (Plinke 2000), possibly because of the material’s high chlorine and additive content (Baitz 2004). If PVC incineration is not executed in accordance with environmental guidelines, the high chlorine content results in the formation of toxic carcinogenic dioxin pollutants at concentrations over three to four orders of magnitude greater than other common plastics (Katami 2002). Dioxins are carcinogenic, and direct exposure to PVC gas results in skin lesions, eye and problems with one’s circulation (Freinkel 2011).
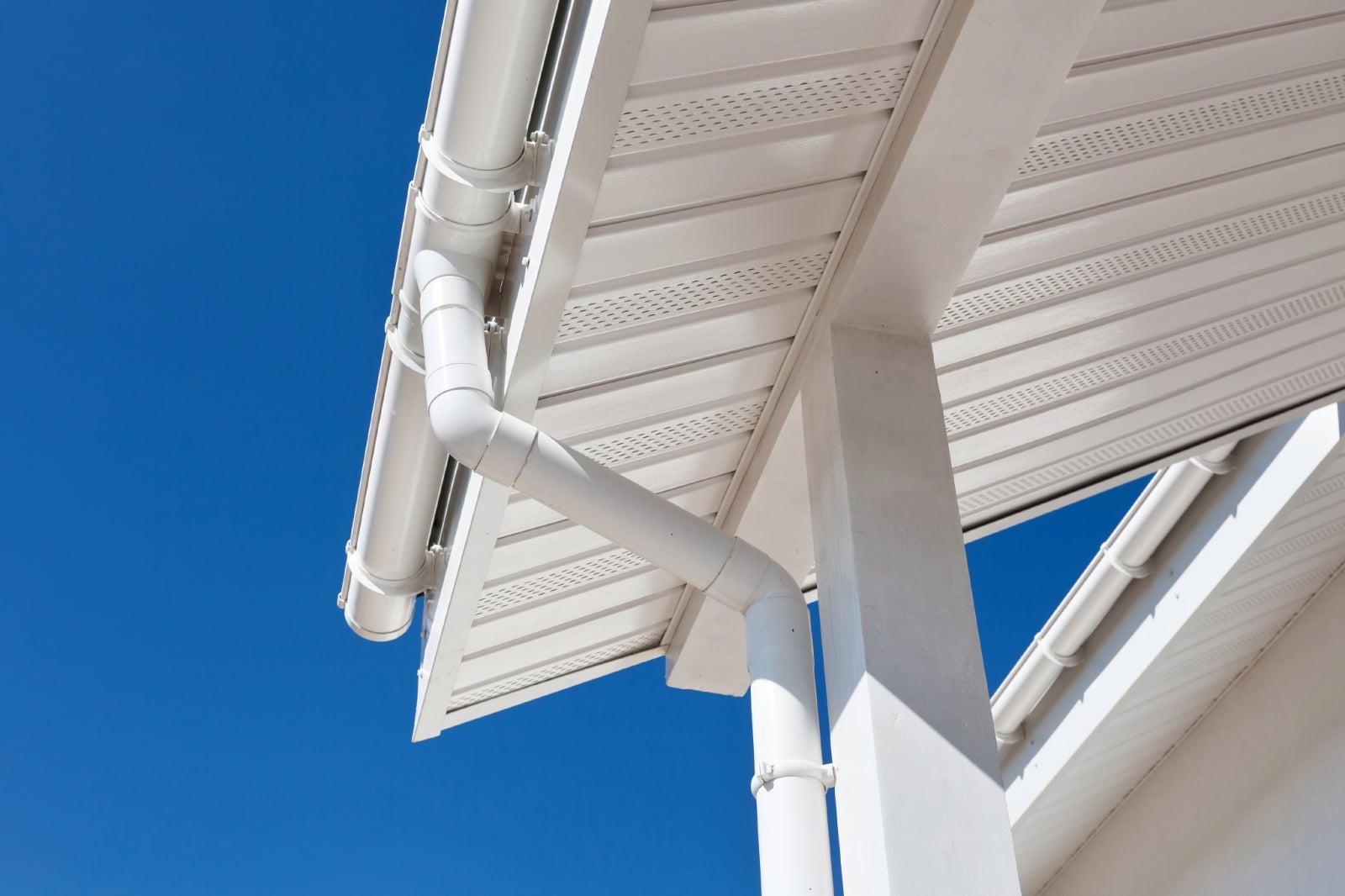
In the United States, EPA regulations protect against dioxin emission from industrial incineration facilities (Venezia 2010); however these restrictions do little to protect the ecosystems and well-being of rural communities that dispose of refuse through “backyard burning.” Such community level incineration is the largest source of domestic dioxin production (NCEA 2006). Moreover, when plastics burn in uncontrolled building fires, the release of dioxins poses serious risks for firefighters and others; as noted in the case of Binghamton State Office Building in 1981 and the World Trade Center fire in 2011 (Fitzgerald et al. 1986, Dahlgren et al. 2007).
Moreover, plastics are with us forever. They may degrade, but they persist. As a result, particulates and microplastics leach and disperse their pollutants in water and air, respectively. As studied by Deonie Allen, of the École Nationale Supérieure Agronomique de Toulouse (ENSAT), particulates no bigger than dust, can be transported and contaminate the air and soil a hundred kilometers from their source (S. Allen, D. Allen et al. 2019).
Hence, whether through leaching, burning, or dispersal, the accumulation of plastic in the human body contributes to increased risks of endocrine disruption that interferes with the proper functioning of estrogen and testosterone, and that has long term effects such as asthma, diabetes, obesity, infertility, and even attention deficient disorder.
These are but a few of the health risks and there are dozens similar materials sourced from fossil fuels that pose significant threats to human health. Their use in the building industry requires a far more integrated Life-Cycle assessment tool that accounts for all risks associated with manipulating different fossil fuel feedstocks, across various material phases (Raw Material Extraction and Transportation, Refining and Chemical Synthesis, Material Manufacturing, Product Fabrication, Product Installation, Building Occupancy and Use, and Waste Management).
For architects, engineers, and builders who seek the ability to evaluate the human health impacts of their material choices, transparency in the sourcing of supply chain data for the most commonly used plastic materials is essential. This is the value of a fully integrated Life-Cycle Index of Human Health in Building (LCI-HHB).
Policy Recommendations
Material Data Disclosure
In most industries, the lack of regulations requiring material disclosure protects suppliers and producers from having to know and communicate the full sourcing of raw materials imbedded in the products they bring to market. The building industry is no different. It is nearly impossible to follow the supply chains of any product introduced in a construction project.
This policy priority maintains that companies should be tasked with reporting on the origins and chemical content of fossil fuel-based materials used in their products so that architects, engineers, builders, and the public can access this information. Product supply companies should fully disclose ALL relevant information required for completing industry specific Material Safety Data Sheets (MSDS). (see Figures 5 and 6)
MSDS documentation is aimed at collecting all data associated with the chemical origins, performance, and possible risks associated with building-based materials. A typical MSDS, calls for information on Chemical product and Company Identification, Composition/Information on Ingredients, Hazards, Fire Fighting Measures, Accidental Release Measures, Exposure Control, Stability and Reactivity, and Toxicology, Ecological and Regulatory Information.
Unfortunately, there are no internationally shared protocols and regulations for completing all sixteen MSDS categories. As a result, designers, consumers, and building occupants have very little confidence that they are fully informed on the nature of materials they come into contact with in the built environment, let alone the full toxicological profile of said materials. Needed is a Material Data Disclosure process for ensuring complete transparency in what concerns the risks associated with the consumption of building based polymers derived from fossil fuels. This policy would be the equivalent of city, state, and federal governments who mandate full disclosure on the amount of energy consumed by buildings.
Material data disclosure has been a priority for a number of key knowledge groups active in this space. The Healthy Building Network, founded in 2000 by Bill Walsh, develops on-line technologies and data structures for promoting the design and construction of healthy buildings (healthybuilding.net). The Network issues a variety of fact-finding reports (including one on the use of chlorines in building products) while making available to the industry important web-based tools. Also working in the space of data disclosure is the Center for International Environmental Policy (CIEL), Earthworks, and UPSTREAM, among others, who published in 2019 “Plastic & Health, The Hidden Costs of a Plastic Planet.” The work is dedicated to naming all phases of the life-cycle of plastic in which humans are placed at risk. It discusses the health impact of handling its raw chemicals, the challenge of using plasticizers and chemical additives, and the larger invisible environmental trauma of micro-particulates forever lost to air and water.
Lastly, the American Institute of Architects (AIA) is also an important player in this space. In spring 2018, it published in collaboration with ARUP “Prescription for Healthier Building Materials: A Design and Implementation Protocol,” which offered strategies for making healthy choices in design. And in the same year it updated the AIA Code of Ethics, with architects now called upon to design “a built environment that equitably supports human health and well-being and is resistant to climate change” (Ethical Standard 2.4). The AIA also offers its members a continuing education Certificate Program on Materials, whose third module is on “Health Impacts: Connecting Building Materials, Human Health, and the Environment.”
The building industry continues to benefit from the leadership shown by these and similar efforts to achieve data disclosure. However, their work remains uncoordinated, independent, and not referenced to a set of integrated metrics. As such, they have yet to achieve the larger policy goals of full material data disclosure of interest to this digest.
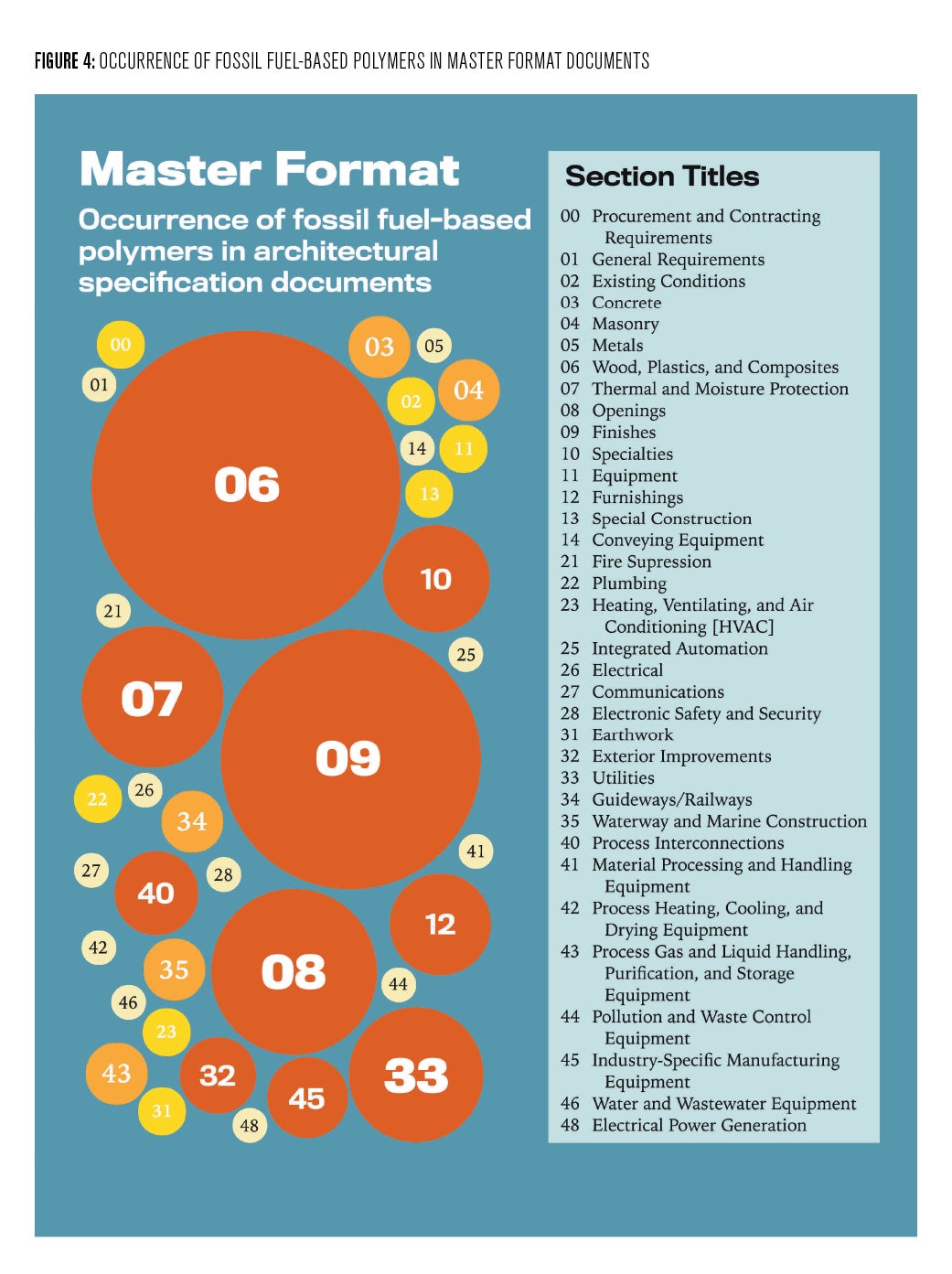
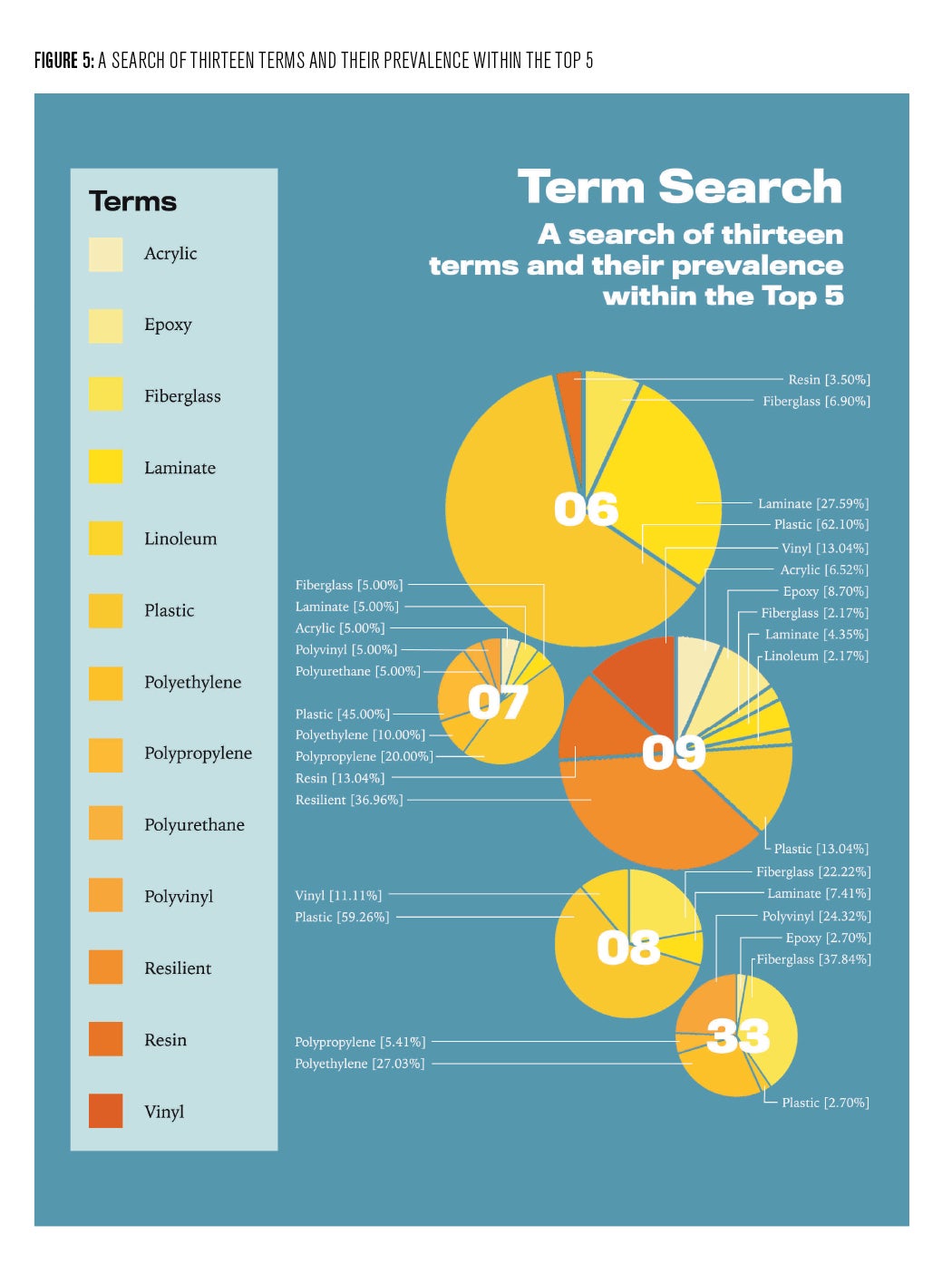
Human Health Risk Education
Professionals involved in delivering building-based services to the public should be educated in their respective disciplines on the subject of material health. University curricula should, at a minimum, introduce language and metrics associated with material toxicology, its delivery mechanisms, and the human health impacts it incurs.
At the moment, American architects are not educated on how materials contribute to air pollution, poor indoor air quality, and environmental toxicity. The National Architectural Accrediting Board (NAAB) makes no mention of human health issues resulting from poor architectural design decisions. Albeit NAAB lists among its “Student Performance Criteria (SPF)” knowledge of the environmental impact of materials, issues of social equity, and the code of professional ethics; there are no Student Performance Criteria that reference the ethical and social justice imperative of delivering “toxic-free” material environments. Changing this is an important policy priority.
Life-Cycle Index of Human Health in Building
This policy priority calls for the development of a protocol for calculating the human health risks associated with the use of fossil fuel-based polymer materials in the building industry. Once disclosure of all chemical sources in building products is possible, significantly more transparency of building material properties and their attendant health risks will be calculatable.
Starting with the Hazards List found in the Data Commons of the Healthy Building Network, an easy-to-use LCI-HHB could be developed for the AEC industry and their clients. This index could help calculate and evaluate the health risks of using fossil fuel sourced building materials across a building’s life and afterlife.
Reporting would involve both toxicity scales and Life-Cycle diagrams. As noted in Figure 4, each moment in the Life-Cycle of a plastic gives rise to a new set of potential risks. The “Waste Management” of PVC, for example, may have risks attendant to demolition, salvaging, and landfill, while transmission pathways may be dermal absorption, ingestion, or inhalation, and the health impacts asthma or eye irritation. The LCI-HHB diagram gives the viewer an easy to read map of where the risks are highest in that plastic’s Life Cycle.
Hence, the Life-Cycle Index of Human Health in Building aims to measure and diagram the health risks associated with each moment in a material product’s life-cycle; its creation being predicated on collaboration by the AEC industry, medical toxicologists, environmental scientists, and material scientists.
In addition, using and distributing the results from such a tool necessitates the development of an easy-to-use digital interface. Architects, engineers, and builders respond best to software tools that are computationally robust during the early stages of a project’s design development and during detailed material selection processes. A recent review of typical LCAs in the building industry characterizes the state of current methodologies as: “fairly fragmented and spread over several national and international publications” (Cabeza 2014).
On the one hand, this level of fragmentation is expected given the incredible complexity of accounting for all possible impacts, and case-by-case approaches should be both expected and encouraged over standardizing a singular impact Index. But this lack of coherence leaves design/build teams without holistic comparative tools for selecting materials and building processes. Developing new methods of analysis and supply chain inventories for each project is capital intensive and improbable (Jensen 1998).
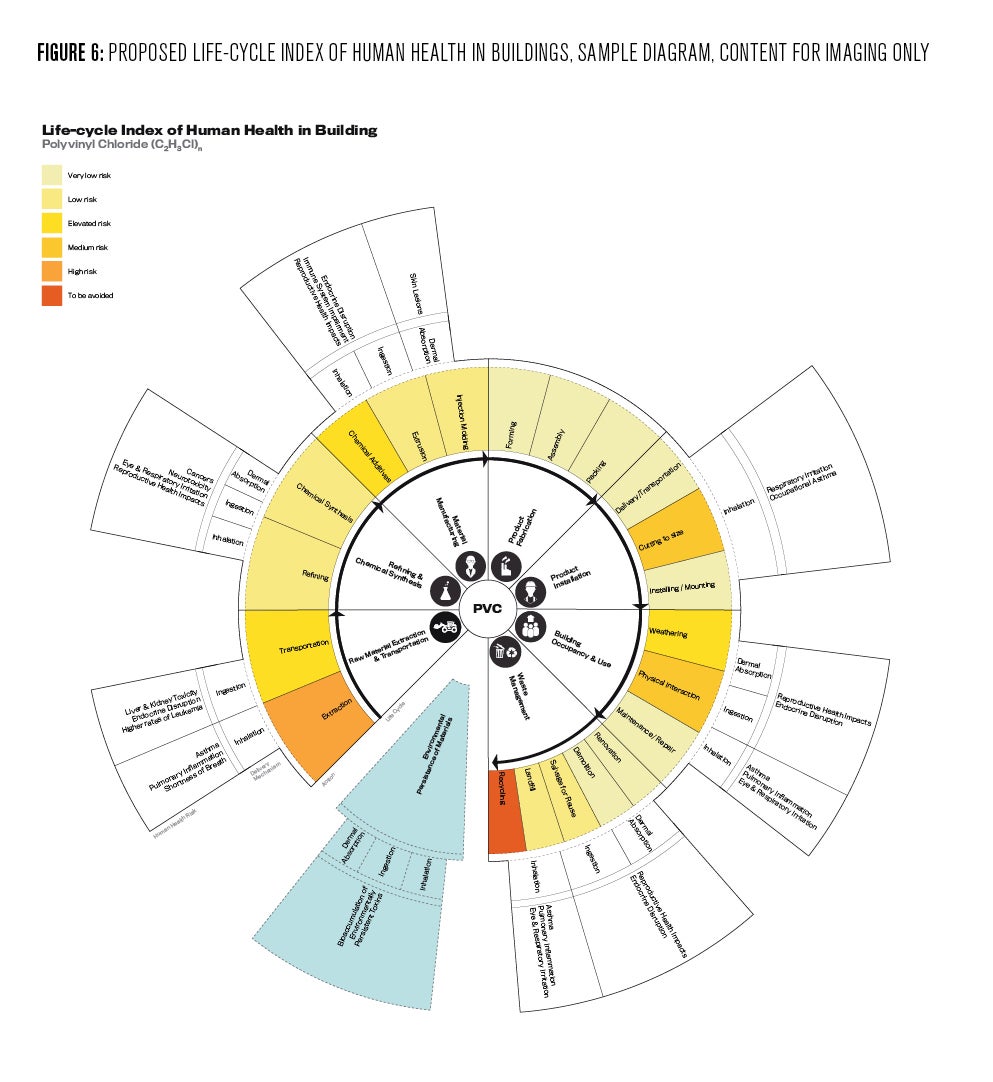
If entire papers are published in order to report on the LCA of two different insulation materials (Papadopoulos 2006), how might we expect to achieve similar detailed evaluations when conducting practice based LCA inclusive of human health factors on an actual building project with hundreds of different material products? As with Tally, where LCA and LCEA tools show early signs of digital integration in design/build Building Information Modeling (BIM) space, so too could human health factors be introduced in an intuitive and integrated software that computes the full risk of material toxicity in building. A robust digital interface for calculating the LCI-HHB can achieve this.
Conclusion
Hence, in conclusion, the policy priorities of Fossil Fuels, the Building Industry, and Human Health, are focused on achieving Material Data Disclosures for all polymers introduced in the building supply chain, updating the education of architects, engineers, and builders in recognizing human health risks of materials, and promoting the creation and deployment of a Life-Cycle Index of Human Health in Building (LCI-HHB).
Franca Trubiano
Associate Professor of ArchitectureFranca Trubiano is Associate Professor of Graduate Architecture and a Registered Architect with l’Ordre des Architectes du Québec.
Chloe Onbargi
Weitzman School of DesignChloe Onbargi is an undergraduate student in the Weitzman School of Design at the University of Pennsylvania.
Antonio Rinaldi
School of Arts and SciencesAntonio Rinaldi is an undergraduate student in the School of Arts and Sciences at the University of Pennsylvania.
Zachary Whitlock
Undergraduate Seminar FellowZachary Whitlock is an undergraduate student in the Vagelos Integrated Program in Energy Research (VIPER) program studying materials science and engineering and environmental science. Whitlock was also a 2020 Undergraduate Student Fellow.
Bayer, Charlene, Russel Gentry, Surabhi Joshi. 2010. “A Guide to Life Cycle Assessment of Buildings in Practice” American Institute of Architects. Washington, DC. https://www.brikbase.org/sites/default/files/aiab082942.pdf
S. Allen, D. Allen et al. “Atmospheric Transport and Deposition of Microplastics in a Remote Mountain Catchment,” Nature Geoscience (2019)
American Chemistry Council. “Major Markets: Building and Construction” 2009. https://plastics.americanchemistry.com/B-C/ Accessed April 4th 2019
Baitz, Martin, Johannes Kreibig, Eloise Byrne, Cecillia Makishi, and Thilo Kupfer. 2004. Life Cycle Assessment of PVC and of Principal Competing Materials. European Commission. Leinfelden-Echterdingen, Germany.
Bjureby, Erika, Mareike Britten, Irish Cheng, Marta Kamierska, and Ernesk Mezak. 2008. The True Cost of Coal: How People and the Planet are Paying the Price for the World’s Dirtiest Fuel. Greenpeace International. https://www.greenpeace.org/archive-international/Global/international/pl… 2/report/2008/11/cost-of-coal.pdf
Building for Environmental and Economic Sustainability (BEES), https://www.nist.gov/services-resources/software/bees and https://ws680.nist.gov/bees/(A(s4j522g11QEkAAAAMTZhMjA2ZDgtYmZkYy00OWJjLTgwYjAtMGUyNjk1NzNkNGRl92eu0lpz1iKrFVoBsvKGUeA4fms1))/default.aspx
Cabeza, Lusia F, Lídia Rincón, Virginia Vilariño, Gabriel Pérez, Albert Castell. “Life Cycle Assessment (LCA) and Life Cycle Energy Analysis (LCEA) of Buildings and the Building Sector: A Review.” Renewable and Sustainable Energy Reviews 29 (2014) 394-416. doi:10.1016/j.rser.2013.08.037.
CIEL. 2019. Plastics & Health: The Hidden Costs of a Plastic Planet. Center for International Environmental Law. Washington, DC. https://www.ciel.org/wp-content/uploads/2019/02/Plastic-and-Health-The-H…
Crompton, TR. 2007. Additive Migration from Plastics into Foods. A Guide for the Analytical Chemist. Smithers Rapra Publishing, Shrewsbury.
Dahlgren, James, Cecchini Marie, Takhar, Harpreet, Paepke, Olaf. “Persistent Organic Pollutants in 9/11 World Trade Center Rescue Workers: Reduction Following Detoxification.” Chemosphere 69 (2007) 1320-1325
Dow Chemical Company. 2019. “Vinyl Chloride Monomer” Product Safety Assessment.
http://msdssearch.dow.com/PublishedLiteratureDOWCOM/dh_08d7/0901b803808d…
Fitzgerald, Edward, Standfast, Susan, Youngblood, Lois, Melius, James, Janerisch, Dwight. “Assessing the Health Effects of Potential Exposure to PCBs, Dioxins, and Furans from Electrical Transformer Fires: The Binghamton State Office Building Medical Surveillance Program.” Archives of Environmental Health 41, n.6 (November/December 1986):368-376.
Franklin Associates, LTD. “Comparative Energy Evaluation of Plastic Products and Their Alternatives for the Building and Construction and Transportation Industries.” (Society of the Plastics Industry, March 1991). https://www.greenbuildingsolutions.org/blog/material-choices-and-resourc…
Freinkel, Susan. Plastic: A Toxic Love Story. (Boston, New York: Houghton Mifflin Harcourt, 2011).
Geyer, Roland, Jenna R. Jambeck, and Kara Lavender Law. “Production, Use, and Fate of All Plastics Ever Made.” Science Advances 3, no. 7 (2017). doi:10.1126/sciadv.1700782.
Katami, Takeo, Akio Yasuhara, Toshikazu Okuda, and Takayuki Shibamoto. “Formation of PCDDs, PCDFs, and Coplanar PCBs from Polyvinyl Chloride during Combustion in an Incinerator.” Environmental Science & Technology 36, no. 6 (2002): 1320-324. doi:10.1021/es0109904
N. Kohler, et al. A Life Cycle Approach to Buildings: Principles-Calculations- Design Tools (DETAIL, Green Books, 2013)
Jensen, Allan A, Kim Christiansen, Anders Schmidt. “Life cycle assessment (LCA): a guide to approaches, experiences and information sources.” Environmental issues, Series no. 6 ed. European Environment Agency, Copenhagen, Denmark; 1998.
Lithner, Delilah, Ildiko Nordensvan and Göran Dave. “Comparative acute toxicity of leachates from plastic products made of polypropylene, polyethylene, PVC, acrylonitrile-butadiene-styrene, and epoxy to Daphina manga.” Environ Sci Pollut Res no. 19 (2012): 1763-1772. doi:10.1007/s11356-011-0663
International Living Future Institute, https://living-future.org/education/life-cycle-assessment-environmental-…
Menzies, F., P.F.G. Banfill. “Lifecycle Assessment and Embodied Energy: A Review.” Proceedings of the Institution of Civil Engineers: Construction materials, no. 160 (2007): 135-143. doi:10.1680/coma2007.160.4.135
Milne, Geoff. Australian Government “Your Home, Australia’s Guide to Environmentally Sustainable Homes,” (2013) http://www.yourhome.gov.au/materials/embodied-energy, Accessed April 25th, 2019.
National Center for Environmental Assessment. 2006. An Inventory of Sources and Environmental Releases of Dioxin-Like Compounds in the United States for the Years 1987, 1995, and 2000. Washington, DC: Office of Research and Development, U.S. Environmental Protection Agency. https://cfpub.epa.gov/ncea/risk/recordisplay.cfm?deid=159286
National Institute of Environmental Health Science. 2010. Endocrine Disruptors. Washington, DC: National Institutes of Health. https://www.niehs.nih.gov/health/materials/endocrine_disruptors_508.pdf
Occupational Safety and Health Administration. 1910.1017 – Vinyl Chloride. Washington, DC. United States Department of Labor. March 2018. https://www.osha.gov/laws-regs/regulations/standardnumber/1910/1910.1017
Papadopoulos, A. M., E. Giama. “Environmental Performance Evaluation of Thermal Insulation Materials and Its Impact on the Building.” Building and Environment 42 (2007) 2178-2187. doi:10.10106/j.buildenv.2006.04.012.
Perkins + Will. Healthy Environments: What’s New (and What’s Not) With PVC. Chicago, IL, (2015). https://perkinswill.com/site/default/filesPerkinsWll_PVC_2015_Whitepaper…
PlasticsEurope. 2017. Plastics – The Facts 2017: An Analysis of European Plastics Production, Demand and Waste Data. https://www.plasticseurope.org/application/files/5715/1717/4180/Plastics…
Plinke, Eckhard, Niklaus Wenk, Gunther Wolff, Diana Castiglione, Mongens Palmark. 2000. Mechanical Recycling of PVC Wastes. Study for DG XI of the European Commission. Plastic Consult, COWI. http://ec.europa.eu/environment/waste/studies/pvc/mech_recylce.pdf
Siemens AG, “Production of Synthetic Rubber, Process Gas Chromatograph MAXUM Edition II Controls and Optimizes Butyl Rubber Production,” (2013); 2.
Simonen, Kate. Life Cycle Assessment (London; New York: Routledge, 2014)
Tasoff. H. “Plastic’s Carbon Footprint, Researchers Conduct First Global Assessment of the Lifecycle of Greenhouse Gas Emissions from Plastics,” The CURRENT, (April 15, 2019) https://www.news.ucsb.edu/2019/019424/plastic-s-carbon-footprint?fbclid=…
USGBC. 2019. “LEED_v4_for_Building_Design_and Construction” Point Breakdown Spreadsheet.
USGBC. US Green Building Council, Buildings and Climate Change
https://www.eesi.org/files/climate.pdf
Venezia, Domenico, Samuel Joshi, Andrew Szurgot, Micheal Van Brunt. 2010 “Energy-from-Waste and Dioxin Emission Control: Is there a Role for PVC Separation” Proceedings of the 18th Annual North American Waste-to-Energy Conference. Orlando, Florida, USA.
Vesterberg, Anna, Magnus Hendermark, Anne-Marie Vass. “PVC in Medical Devices: An Inventory of PVC and Phthalates Containing Devices Used in Health Care.” Department of the Environment and Sustainability. Karolinska University Hospital. Stockholm, Sweden. 2005
Zheng, J. and Suh, S. “Strategies to Reduce the Global Carbon Footprint of Plastics” Nature Climate Change 9, 374-378 (2019)