Feasibility of Seasonal Storage for a Fully Electrified Economy
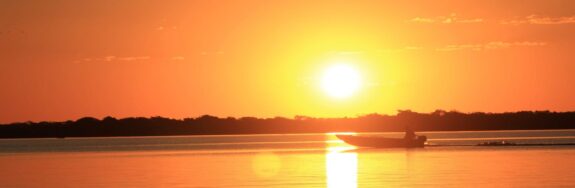
Building electrification is often viewed as a key strategy for transitioning away from fossil fuels. However, at very high levels of renewable deployment, heating electrification's impact on seasonal demand can result in severe load balancing issues.
This research was made possible through a generous gift from Carl Goldsmith (W’88).
The “electrify everything” strategy for transitioning to a clean energy system is, in many ways, extremely logical. Wind turbines and photovoltaic solar cells are the cheapest and safest technologies we currently have for generating carbon-free energy, so it makes sense to shift as much of our energy demand as possible to the electricity grid. However, this strategy for decarbonization presents the considerable challenge of how to store vast quantities of electrical energy. Solar and wind power are inherently variable over the course of hours, days, weeks, seasons, and years, and as our economy becomes increasingly electrified, demand variation will intensify.
There are numerous storage technologies available, but most are still too inefficient or expensive to be deployed for large-scale load-balancing. Even those that have been successfully deployed are only meeting a small fraction of the storage capacity that would be demanded by a fully-electrified and renewably-powered grid (Cardwell 2017).
Last year, the Kleinman Center published a report outlining possible future responses available to Philadelphia Gas Works (PGW) when faced with the imposition of a federal or state carbon price—something that is looking increasingly likely over the coming years (Serpell et al. 2019). That report explored two possible strategies for fully decarbonizing the heating demand of 500,000 Philadelphia customers who currently rely on pipeline-delivered natural gas.
One option would be for customers to electrify this energy demand by replacing behind-the-meter appliances such as furnaces and cookstoves. The other option would be for PGW to replace the supply of natural gas with synthetically manufactured methane using renewably powered electrolysis and direct-air-capture of CO2.
While both proposed strategies prove to be extremely expensive because of the huge energy demand that was being decarbonized, the electrification strategy turns out to be significantly more affordable. However, the scope of the PGW report did not allow for an in-depth analysis of the additional storage demand that the electrification strategy would put on the grid.
In an effort to refine the cost estimates made in the PGW report, and to expand the scope of our most recent findings, this digest will specifically tackle the potential impact of electrified heating on the generation and storage demands of the electricity grid within the PJM footprint.
Seasonal Demand for Storage Capacity
The fundamental challenge explored by this digest is the increased seasonal variability of electricity demand that is created by electrifying space and water heating. Currently in the PJM interconnection, seasonal electricity demand peaks in the summer (see Figure 1).
However, our analysis shows that electrifying natural gas and fuel oil demand for space and water heating would cause wintertime electricity demand to spike, reversing and worsening the seasonal variation that is currently experienced by the grid. It is likely that throughout much of the PJM footprint, air-conditioning demand will also increase in the summer months as the warming effects of climate change worsen, but this will still yield more extreme fluctuations between seasons as demand drops in the spring and fall.
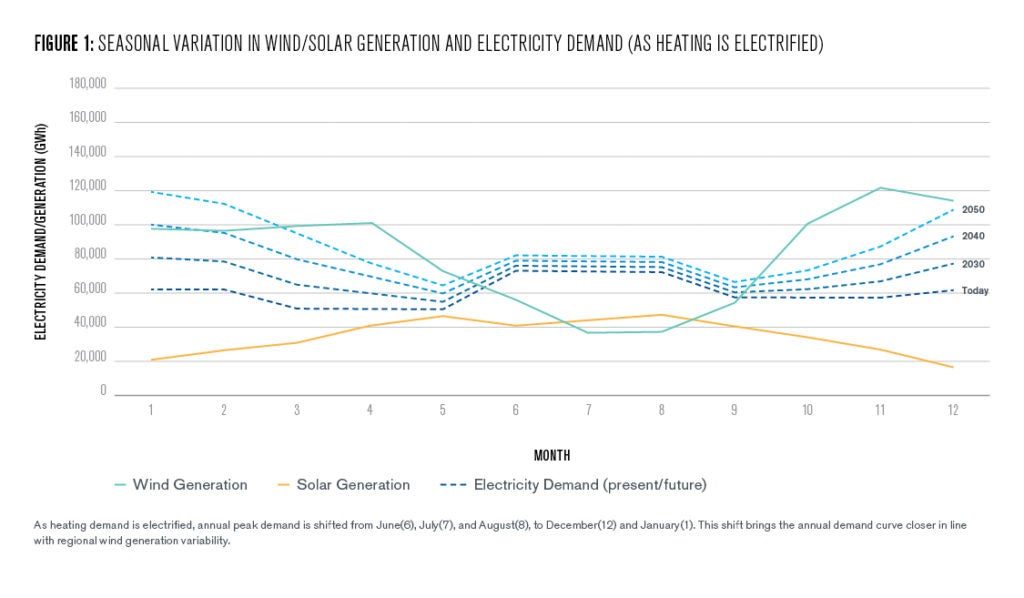
Furthermore, the energy capacity demanded from storage increases the longer the load balancing timeframe becomes, and the per-cycle cost of operating storage assets increases linearly. To better understand this concept, consider the famous duck curve—the shape of daily demand in a heavily solar-dependent system.
Supply of solar is at its peak in the middle of the day, but demand for energy peaks in the evening. In this system, daily load balancing can be delivered by enough storage to shift one day’s worth of excess supply to one evening’s worth of excess demand. However, if you extend this pattern of load shifting to a week, month, or year, with just one period of excess supply, and one period of excess demand, the amplitude of that load shifting increases considerably and the number of annual cycles decreases.
In the following sections, we explore three available technologies for long-duration, seasonal storage: Reversible fuel cells coupled with hydrogen storage, lithium-ion batteries, and pumped hydroelectric storage. These three storage technologies were selected for comparison because of their relative viability and practicality.
Three critical parameters are chosen for comparison: land-use, electrical efficiency (storage input vs output), and cost. The land mass required for each storage scheme is calculated from the energy density of the storage technology, while costs are representative of minimum and maximum estimates for current prices.
Reversible Hydrogen Fuel Cell
The reversible hydrogen fuel cell (RHFC) is a technology that converts electricity into hydrogen gas that can later be used to generate electricity when needed. In many ways, this technology can be thought of as a mimicry of several chemical energy storage systems found in nature, such as plant photosynthesis which uses solar energy to convert water and CO2 into sugars that are then stored for later use.
RHFC systems require three components: a water electrolyzer that splits water into hydrogen and oxygen using electricity, a hydrogen gas compression and storage facility, and a hydrogen fuel cell which generates electricity from hydrogen. The total electrical efficiency of RHFCs—typically in the range of 30% to 35%—is derived from the individual efficiencies of the systems three primary components. (Schoenung, 2011 and Pellow et al., 2015).
Recent advances in compressed hydrogen storage on automobiles have achieved energy densities up to 880 kWh/m3. Thus, a hypothetical storage facility with 40 m height will require 0.007 acres of land per GWh. Overall, costs of $150 to $575/kWh have been estimated by Sandia National Lab for a 20-year RHFCs storage scheme. This considerable cost range is illustrative of RHFC technology’s immaturity, with very few practical applications from which to estimate costs.
Li-ion
While several types of batteries are currently employed in various products, lithium-ion batteries have become extremely popular because of their high energy density and applicability for large scale storage.
Electrons supplied by electrical input are stored in lithium-ion batteries as lithium ions leave the positive electrode and travel through an electrolyte to a lithium-graphite negative electrode. When connected to a circuit, electrons flow in the opposite direction of the lithium ions. When electricity is needed from the battery, this compound breaks down into lithium-ions and electrons to create electrical output.
This simple and reversible process results in very little loss of energy and has an efficiency of around 95% (PowerTech, n.d.). The energy density of lithium-ion batteries depends on the type of lithium compound, lithium-ion salts, and electrolytes used and is slightly lower than that of compressed hydrogen. Assuming a 40-meter height, an estimated 0.58 acres of land is required per GWh (Tesla, n.d.).
Current capital costs of Li-batteries range from $175 to $520/kWh, with an expected lifetime of approximately eight years (Lazard 2018). Because the expected lifetime of the highlighted technologies differ considerably, cost/kWh estimates were adjusted to cost/kWh/year estimates.
Pumped Hydroelectric Storage
Pumped hydro storage accounts for 96% of grid-scale energy storage in use today. It uses excess grid electricity to pump water from a lower reservoir to a higher reservoir (Kenning 2017). When energy demand increases during peak periods, the water can be released to drive a turbine for electricity generation as it flows back to the lower reservoir under the force of gravity.
Pumped hydro storage is a mature technology that has been commercialized for decades with a moderate efficiency of up to 80% (Luo et al. 2015). However, the land requirement is substantial. An analysis of 14 different existing and pending pumped hydro storage facilities in the eastern United States revealed an average land use of 133 acres per GWh.
Pumped hydro storage also varies significantly in cost, from $5 to $100/kWh depending on the natural geography of the construction site (Kouskou et al. 2014). Even at the top end of this range, it the most affordable of the three storage solutions that are discussed, at least in terms of energy density.
Cost Optimization Model
In order to determine the likely cost implications of various storage technologies to a zero-carbon grid, we designed a simple Python model that calculates the optimal balance of solar, wind, and storage deployment under a range of independent variables in order to minimize cost to the end user. The model also provides the total acreage of land needed for deployment (including land used for solar and wind power), and the maximum energy supplied by storage in the highest demand month.
Using this model, we calculated the optimal deployment and impact on cost and land area of the three different storage technologies under two different grid scenarios; one in which all heating demand continues to be met by natural gas and fuel oil, and a test scenario in which all heating is electrified. By comparing these two scenarios, we were able to estimate the additional cost to end users of a fully electrified heating system.
To ensure that both grid scenarios were relevant for future policy considerations, we assumed a 100% clean generation mix in both grid scenarios. Because PJM depends on nuclear, another carbon-free energy source, for 15% of its generation, this capacity was maintained in the model.
All remaining demand was met by an optimal mix of wind, solar, and storage. The model scenarios assume that operators will be compensated via wholesale electricity prices to build enough storage to deal with a high energy demand scenario but does not make any assumptions about how this pricing structure would operate (Sankar 2019).
Model Results and Limitations
Under most cost and demand parameters, the model opted to significantly overbuild solar and wind generation capacity to avoid considerable dependence on storage resources during peak interseasonal demand, and only deployed the minimum daily storage required by the model to accommodate daily load variability. Pumped hydro storage was the one exception to this rule.
Under the lowest cost parameters, interseasonal pumped hydro storage was deployed and largely displaced the redundant generation capacity used in other scenarios. This further demonstrates the considerable cost of deploying storage that may only be used intermittently throughout the year.
Another significant finding of the optimization model is that when peak interseasonal demand is shifted to the winter by electrified heating, the model strongly favors increased deployment of wind capacity over solar. This yielded a much larger land footprint for the electrified heating scenario over the base case. In some runs of the model, the land demand for solar and wind is similar in size to the state of Virginia, almost certainly exceeding regional limits unless relying heavily on off-shore wind.
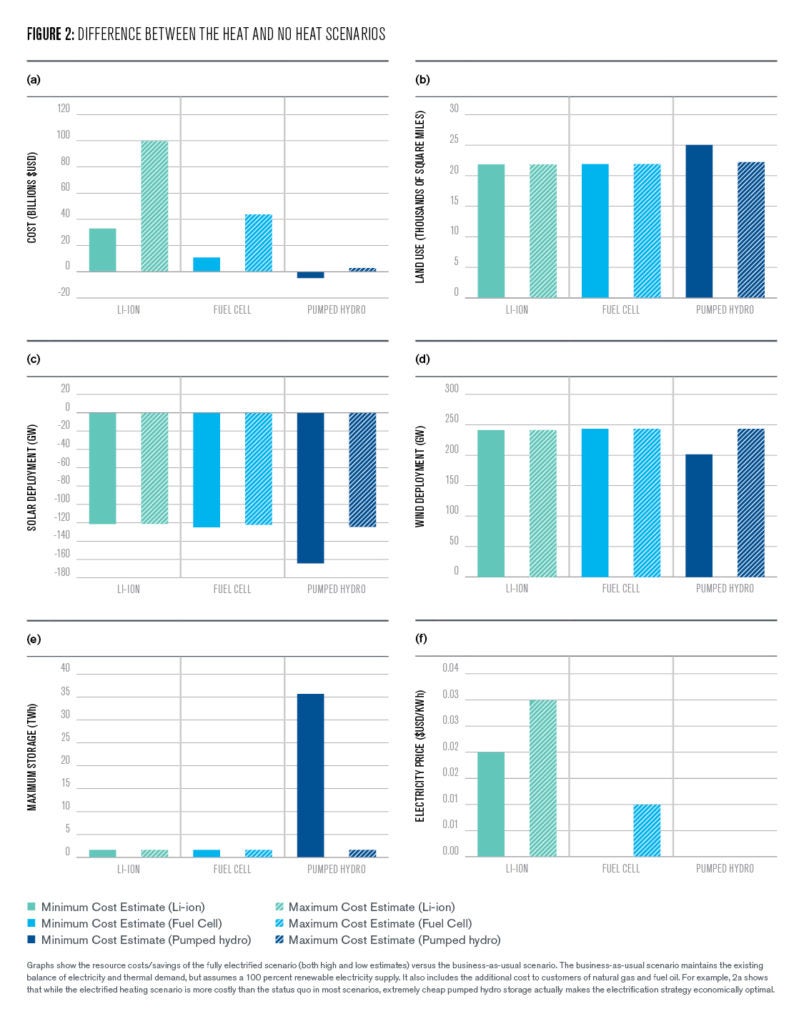
A key limitation of the model is that it assumes perfectly predictable and regular interseasonal heating demand based on the average historical heating demand in the PJM region. In reality, there will be significant yearly variation in both the renewable supply (amount of wind and sun during the winter—see Figure 3) and the heat demand (based on winter temperatures). Given how difficult it is to predict electricity demand and weather over short timeframes, accurately predicting weather and demand three to six months ahead of time will be an immense challenge for operators and will likely require the further over-deployment of storage or generation to prevent energy shortages.
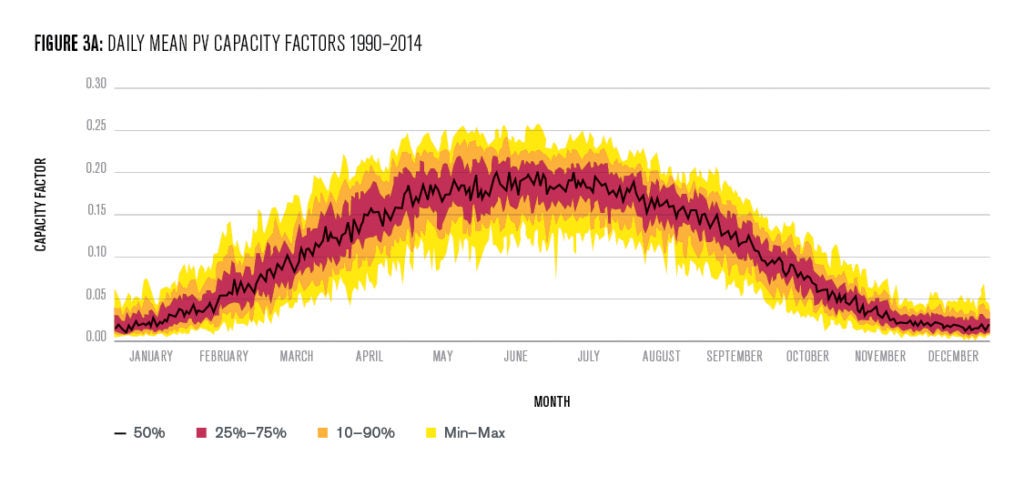

Cost Implications for PGW Decarbonization Strategies
The PGW report released by the Kleinman Center in 2019 concluded that although the electrification strategy required a much higher capital investment (10.7 billion vs 4.92 billion $USD) the higher electricity demand of the methanation strategy to meet the same heating demand (43,000 GWh/year vs. 22,848 GWh/year) meant that the methanation strategy resulted in much higher energy costs for Philadelphia customers (Serpell et al. 2019). In order for PGW’s natural gas prices to match the per household costs of the electrification and methanation strategies, a carbon price of $104 and $381 per ton of CO2e, respectively, would need to be imposed on the utility.
The model designed for this digest significantly alters the finding of the PGW report by incorporating a standard transmission and distribution cost based on historical data from PJM and by embedding the cost of new storage required to balance zero-carbon, variable generation.
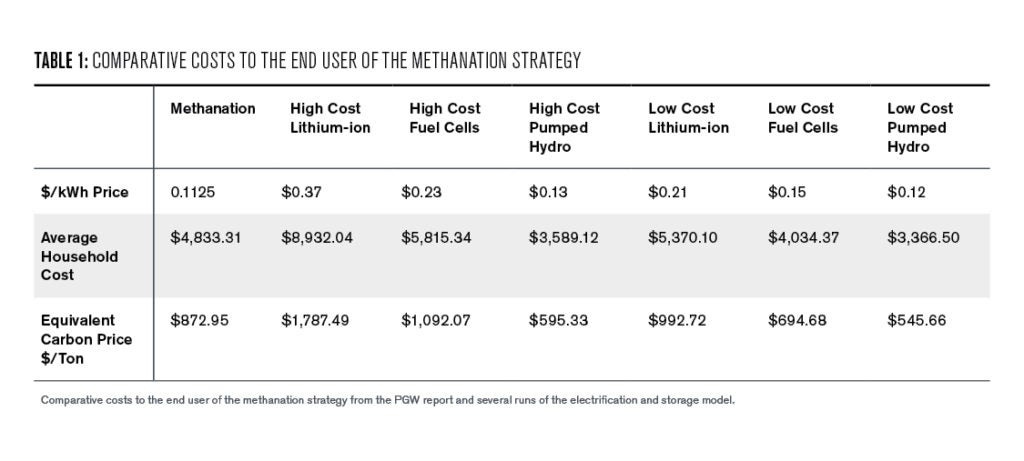
By modeling the optimized grid with and without electrified heating, the minimum electricity costs for customers of the electrification strategy were quantified. These costs were then incorporated into the existing PGW decarbonization strategies to update their costs and equivalent carbon prices. Costs for the methanation strategy were updated to include distributional cost but not the cost of additional storage, as this strategy has an inherent storage method in the form of synthetic methane.
Once full delivery costs were incorporated into the methanation strategy, the equivalent carbon price increased from $381/ton to $873/ton, an increase attributable to the strategy’s enormous electricity demand. Meanwhile when assuming high costs of storage for the three storage technologies considered by the model, the equivalent carbon price for the electrification strategy was between $595 to $1,787/ton depending on the storage technology that was used. This represents a shocking 6 to 18x increase over the costs described in the PGW report. Under more optimistic low-cost estimates of storage, the equivalent carbon price for the electrification strategy ranged from $545 to $993/ton.
Comparison of Different Storage Methods
The above analysis points to pumped hydro storage being the most cost-efficient option for meeting seasonal storage demand. This can be attributed to the longevity of operating facilities (up to 50 years) and the mature and low-cost technologies that are used (hydro pumps and turbines). That said, pumped hydro storage is significantly limited by geographical requirements. It requires a significant elevation gradient and enough space for two large reservoirs.
Within the PJM footprint, this limits the technology to regions with vast unused lands and natural variations in elevation such as parts of Appalachia. This model did not limit the land availability for pumped hydro and did not assume any additional transmission costs, however future analysis should likely find a way to incorporate regional land limitations.
RHFC technology costs significantly more to deploy than pumped hydro but is not restricted by geography. Out of the three storage schemes considered, RHFC requires the least amount of land per kWh.
That said, our calculations are based on facilities that store hydrogen at 700 times ambient atmospheric pressure. Though this has been presented as a reasonable storage pressure for onboard hydrogen in zero-emission vehicles, the amount of hydrogen that is needed for seasonal storage presents an incredible safety risk when stored at such high pressures due to its flammability. Much more research is needed to address the low efficiencies and safety requirements of RHFCs.
Although lithium-ion batteries are the most expensive storage technology in our analysis, technological advancements are fueling a rapid decrease in costs, they have the highest efficiency out of the three storage schemes considered, and they are scalable and can be used in almost any location.
Policy Recommendations
Regardless of the storage technology that is used or the grid scenario that is assumed, the optimization model consistently opts to over-build renewable capacity before relying on storage capacity to balance load. This is because the energy demanded from storage to meet seasonal peak power is so considerable that the cost of deploying storage is far more expensive than building additional wind and solar generation to reduce the peak demand for storage.
This over-deployment of generation capacity means that the cost-optimal scenarios have a land use demand that very likely exceeds reasonable regional limits. In future analyses, the optimization tool ought to incorporate a rising cost of land in order to represent the limits of the PJM region.
Based on this analysis, pumped hydro appears to be the only cost-viable storage option, and only under the most ambitious cost assumptions. Under optimistic technology cost assumptions, renewable electrification of heating within the PJM footprint coupled with pumped hydro storage costs less than meeting the business as usual energy demand with 100% renewable electricity and synthetic natural gas.
In addition, electrified heating using low cost fuel cell technology and high cost pumped hydro technology was competitive with fossil fuel heating even though storage was not widely deployed. This indicates that the required overbuilding of wind capacity to meet electrified heating needs was still more economical than maintaining the fuel costs of fossil fuel heating and decarbonizing the existing electricity grid. Lithium-ion batteries are not currently economically viable even under optimistic cost conditions.
The impact of these model outputs on the final cost calculations for the decarbonization of PGW demonstrates that balancing an all-encompassing, renewably-powered grid carries significant costs that go well beyond the storage costs of hourly or daily load balancing.
Electrifying heating significantly increases interseasonal demand variability, requires significant over-deployment of renewable generation, and demands storage solutions that are only fully charged or discharged intermittently throughout the year. This presents a fundamentally different challenge than simply balancing load-variation on an hourly or daily basis for which power delivery, not energy density, is of primary importance and which is inherently much more limited in its overall demand.
Exactly how an extended and renewably-powered grid would be balanced will ultimately be determined by technology cost curves over the next decade or more. In the meantime, policy makers should acknowledge that simply “electrifying everything” is not in and of itself a viable strategy for building a resilient and low-carbon energy system.
Furthermore, this analysis demonstrates the importance of maintaining baseload power even in a decarbonized grid. Despite environmental and public safety concerns, policy makers may have no choice but to selectively deploy zero-carbon generation sources other than solar and wind such as hydro power, nuclear, and fossil fuel combustion with carbon capture and sequestration/utilization.
Lastly, although the costs of renewably supported electrification are much more considerable than initially calculated in the PGW report, the cost of alternative decarbonization strategies also increases considerably. Even with the need to manage inter-seasonal supply and demand variability, electrifying heat is still relatively cost competitive with synthetic fuel replacement depending on the storage technologies and cost assumptions that are used.
Future refinement of this analysis should likely consider the load balance and storage implications of system wide electrification including industry and transportation. It is likely that an overall increase in demand may smooth out the seasonal variation of heating demand and reduce the overall storage to generation ratio.
For more information on the model used in this research, please contact Oscar Serpell at serpello@upenn.edu.
Oscar Serpell
Deputy DirectorOscar Serpell oversees all student programming, alumni engagement, faculty and student grants, and visiting scholars. He is also a researcher, writer, and policy analyst working on research initiatives with students and Center partners.
Wan-Yi “Amy” Chu
Assistant Professor, Mills CollegeWan-Yi “Amy” Chu is a former postdoctoral researcher in the Goldberg Group, located in the Department of Chemistry at the University of Pennsylvania.
Benjamin Paren
Alumni Research FellowBenjamin Paren is an alumni research fellow at the Kleinman Center and a postdoctoral research associate in the Research Laboratory of Electronics at the Massachusetts Institute of Technology.
Giridhar Sankar
The Wharton SchoolGiridhar Sankar is currently pursuing a master’s of business administration from the Wharton School and a master’s of international studies from the Lauder Institute.
Cardwell, D. 2017. “Tesla Gives the California Power Grid a Battery Boost.” The New York Times. Accessed 01/07/20: https://www.nytimes.com/2017/01/30/business/energy-environment/battery-storage-tesla-california.html
Center for Sustainable Systems, University of Michigan. 2019. “U.S. Grid Energy Storage Factsheet.” Pub. No. CSS15-17. Accessed 01/07/20: http://css.umich.edu/sites/default/files/US%20Grid%20Energy%20Storage_CSS15-17_e2019.pdf
IEA (International Energy Agency). 2014. “Technology Roadmap, Energy Storage.” Accessed 01/07/20 https://speicherinitiative.at/assets/Uploads/20-technologyroadmapenergystorage.pdf
Jaskula, B.W. 2017. “Lithium Mineral Commodity Summary.” United States Geological Survey. Accessed 01/07/20: http://prd-wret.s3-us-west-2.amazonaws.com/assets/palladium/production/atoms/files/mcs2019_all.pdf
Kenning, Tom. 2017. “EnergyAustralia Ponders World’s Largest Seawater Pumped Hydro Energy Storage Plant.” Energy Storage News. Accessed 01/07/20: https://www.energy-storage.news/news/energyaustralia-ponders-worlds-largest-seawater-pumped-hydro-energy-storage
Kouskou, T., Bruel, P., Jamil, A., El Rhafiki, T., Zeraouli, Y. 2014. “Energy Storage: Applications and Challenges.” Solar Energy Materials & Solar Cells 120, 59-80. Accessed 01/07/20: https://www.academia.edu/11481095/Energy_storage_Applications_and_challenges
Lazard. 2018. “Lazard’s Levelized Cost of Storage Analysis—Version 4.0.” Accessed 01/07/20: https://www.lazard.com/media/450774/lazards-levelized-cost-of-storage-version-40-vfinal.pdf
Luo, X., Wang, J., Dooner, M., & Clarke, J. 2015. “Overview of Current Development in Electrical Energy Storage Technologies and the Application Potential in Power System Operation.” Applied Energy 137, 511–536. Accessed 01/07/20: https://www.sciencedirect.com/science/article/pii/S0306261914010290
McLaren, J. 2016. “Batteries 101 Series: How to Talk About Batteries and Power-to-Energy Ratios.” National Renewable Energy Laboratory. Accessed 01/07/20: https://www.nrel.gov/state-local-tribal/blog/posts/batteries-101-series-how-to-talk-about-batteries-and-power-to-energy-ratios.html
Pellow, M., Emmott, C., Barnhart, C. Benson, S. 2015. “Hydrogen or Batteries for Grid Storage? A Net Energy Analysis.” Energy and Environmental Science 8, 1938-1952. Accessed 01/22/20: https://pubs.rsc.org/en/content/articlelanding/2015/EE/C4EE04041D#!divAbstract
Pfenninger, S. 2017. “Dealing with Multiple Decades of Hourly Wind and PV Time Series in Energy Models: A Comparison of Methods to Reduce Time Resolution and the Planning Implications of Inter-Annual Variability.” Applied Energy 197, 1-13 Accessed 01/07/20: https://www.sciencedirect.com/science/article/pii/S0306261917302775
PJM. 2018. “The Value of Markets.” Accessed 01/07/20: https://www.pjm.com/-/media/about-pjm/newsroom/fact-sheets/the-value-of-pjm-markets.ashx
PowerTech. n.d. “The Lithium Difference.” Accessed 01/07/20: https://www.powertechsystems.eu/home/tech-corner/lithium-ion-battery-advantages/
REN21. 2017. Renewables 2017 Global Status Report. Accessed 01/07/20: http://www.ren21.net/gsr-2017/
Roberts, D. 2017. “The Key to Tackling Climate Change: Electrify Everything.” Vox. Accessed 01/07/20: https://www.vox.com/2016/9/19/12938086/electrify-everything
Sankar, Girish. 2019. “Building More Energy Storage Means Building the Right Incentives.” The Kleinman Center for Energy Policy. Accessed 01/22/20: https://kleinmanenergy.upenn.edu/blog/2019/12/17/building-more-energy-storage-means-building-right-incentives
Schoenung, S. 2011. “Economic Analysis of Large-Scale Hydrogen Storage for Renewable Utility Applications.” Sandia National Laboratories. Accessed 01/22/20: https://prod-ng.sandia.gov/techlib-noauth/access-control.cgi/2011/114845.pdf
Serpell, O., Chu, W., Paren, B., Sankar, G., 2019. “Preparing PGW for a Low-Carbon Future.” The Kleinman Center for Energy Policy. Accessed 01/07/20: https://kleinmanenergy.upenn.edu/paper/preparing-pgw-low-carbon-future
Tesla. n.d. “Meet Powerwall, Your Home Battery.” Accessed 01/07/20: https://www.tesla.com/powerwall
Wilson, G., Taylor, R., Rowley, P. n.d. “Heat Decarbonization Challenges: Local Gas vs Electricity Supply.” UK Energy Research Centre. Accessed 01/07/20: http://www.ukerc.ac.uk/publications/local-gas-demand-vs-electricity-supply.html