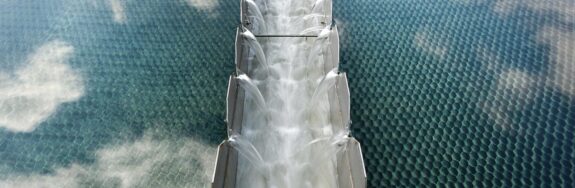
The cost of desalination is falling rapidly, but energy needs are still high. Renewables are critical to making desalination sustainable. More R&D investment and cost support is essential to solving the water-energy nexus.
If there’s one near-inexhaustible resource on Earth, it’s the saltwater that covers two-thirds of the globe. For decades, water-scarce regions have looked to the sea as an almost unlimited water source.
But desalination, the process of removing salt to make drinkable water, is extremely energy-intensive and has long been seen as too expensive for all but niche applications. Can desalination ever mean an end to thirst? New technology and changing economics have made desalination more attractive. Yet the basic challenge remains the same: breakthroughs in energy production and storage are needed for desalination to help make global development more sustainable.
The Promise of Desalination
There are many sources of water for the world’s cities, farms, and factories. Most of the world’s agriculture relies on rainfall, while its cities and industries depend mostly on a combination of surface water from streams, rivers, and reservoirs. Groundwater is an important source of water for agricultural, industrial, and urban water users. But each of these water sources can be unpredictable, especially under climate change.
Only one potential source of water, the sea, offers the promise of completely breaking with the hydrological cycle, and offering a consistent supply of fresh water irrespective of variation in rainfall and water availability. For this reason, highly water-scarce areas have long looked to desalination technologies to supply drinking water. The first practical desalination plant was built on the island of Curacao in 1928, and the first large-scale one opened a decade later in Saudi Arabia (National Research Council, 2008).
Today, global desalination capacity exceeds 86 million cubic meters per day. While this amounts to only about one percent of the world’s drinking water, the global desalination market is on track to grow by an average of approximately 9% in the entire twenty-eight-year period between 1990 and 2018 (Voutchkov, 2016); (Ziolkowska & Reyes, 2016).
Desalination capacity is overwhelmingly concentrated in water-scarce countries in the Middle East and North Africa (see Figure 1). A few countries in this region, like Israel, depend on desalinated seawater for more than half of their total water supplies (U.N. Food and Agriculture Organization, 2016). Even for these highly water-stressed countries, however, desalinated water is almost entirely produced for domestic and municipal use, as it remains far too expensive for irrigation (Reif & Alhalabi, 2015).
The Costs of Desalination
Most of this rapid growth for drinking water supply has been driven by steadily declining costs for key desalination technologies, combined with the growing cost of alternative water sources, especially given more frequent droughts. The cost of reverse-osmosis (RO) technology, the most popular method for large- and medium-scale seawater desalination, has fallen especially fast. RO involves forcing water across a semi-permeable membrane to remove salts, and has become increasingly popular for seawater desalination because of its relatively low energy requirements compared to distillation and other means of removing salt from seawater.
Total costs for RO are approximately 35% each for capital and energy, 12% for the chemicals needed to purify desalted water to meet necessary standards, 6% maintenance, and 5% filters and membranes, with the remainder consisting primarily of labor costs (National Research Council, 2008). Recent estimates from the International Water Association, an industry group, predict significant decreases in construction costs for seawater RO (see Figure 2).
The cost of desalinated water production remains highly variable around the globe, but one of the most advanced plants, Israel’s Sorek, sells water to utilities at US$0.58 per cubic meter, as compared to a global average of approximately US$0.8 (Talbot, 2015). But while these trends are likely to continue to improve the economics of seawater RO desalination, it remains roughly twice as expensive as alternatives like expanded use of groundwater (Badiuzzaman, McLaughlin, & McCauley, 2017).
The primary source of this continued cost discrepancy is the large amount of energy required for seawater RO. Because RO involves forcing water through a semi-permeable membrane to remove salts, there are thermodynamic limits to the efficiency of the process. Energy requirements are approximately 3 to 7 kWh/m3 for seawater desalination, and in practice 35 to 60% of source water can be efficiently recovered as freshwater using current technologies. While pre-treatment, energy recovery, and other techniques can push the energy efficiency of the process to 74% under ideal conditions, this is viewed as a ceiling.
Newer membrane technologies offer some potential to realize significant energy savings. For example, biomimetic membranes, which mimic cellular membranes by selectively filtering out salt and other undesired substances, may reduce the energy requirements of existing membrane filtration. Yet, even with these new membranes, it is unlikely that revolutionary improvements in energy efficiency can be realized (National Research Council, 2008); (Semiat, 2008). These high energy requirements, and accompanying costs, remain the single biggest barrier to expanded use of desalination.
Making Desalination Sustainable
Apart from the direct costs of energy required for seawater desalination, associated environmental impacts remain one of the greatest concerns regarding its expanded use. Many of the direct environmental impacts of desalination plants can be mediated through design or relatively simple technological adjustments. Burying intake pipes in sand, for example, can almost eliminate the problem of ingesting fish and other marine organisms, while devices that diffuse desalination plant outflows can help ensure that brine discharge does not disrupt local habitats (National Research Council, 2008).
But no such ready technological fix exists for conventional desalination plants to reduce the high greenhouse gas emissions associated with large-scale desalination. Estimates suggest that seawater desalination generates 1.5 to 2.4 times as many greenhouse gas emissions as long-distance surface water transport, though such estimates are highly sensitive to energy intensity measures (Stokes & Horvath, 2009).
For countries highly dependent on desalination, the associated greenhouse gas emissions can be very substantial (Kajenthira, Siddiqi, & Anadon, 2012). Desalination plants account for a third of the United Arab Emirates’ total emissions, for example, and globally desalination plants are estimated to be responsible for 76 million tons of carbon dioxide emissions annually, a number that is expected to grow to 218 million tons by 2040 (MENA Report, 2016); (Steel, 2017).
For this reason, growing attention has been paid to the idea of powering desalination with renewable energy sources. In principle, a number of unconventional energy sources can be coupled with desalination, including nuclear, wind, and geothermal. Most of these, however, face seemingly insurmountable economic obstacles. Waste heat from nuclear power generation is already used to power small-scale (3000 cubic meters per day capacity) desalination facilities in Japan. Yet capital costs for nuclear-powered desalination are estimated at being four times as high as fossil-fueled alternatives, while operation costs are estimated to be double (International Atomic Energy Agency, 2000); (Stieglitz & Docksai, 2009).
The technical feasibility of wind-powered desalination has been demonstrated in favorable geographies like isolated small islands. However, costs remain extremely high, in part because the problem of intermittency wherein power generation capacity fluctuates considerably and is especially the case for wind power (Carta, Gonzalez, & Subiela, 2003); (Maleki, Pourfayaz, & Ahmadi, 2016); (Loutatidou, Liosis, Pohl, Ouarda, & Arafat, 2017). While optimal siting, including offshore floating desalination plants, and next-generation battery storage may help overcome these issues, the economics are likely to continue to be unfavorable for wind-powered desalination (Steel, 2017).
As a result of these limitations, among renewable power sources, solar has received by far the greatest attention from researchers. Two forms of solar power have been proposed as a power source for medium- and large-scale desalination: photo-voltaic (PV) and solar-thermal. From a technical perspective, solar-thermal technologies like parabolic trough collectors and central receiver power plants are attractive, because they generate heat that can be used directly for desalination, rather than first generating heat using electricity as with PV technologies (Fiorenza, Sharma, & Braccio, 2007); (Reif & Alhalabi, 2015).
Solar-thermal technologies face no significant technical obstacles to greater adoption. However, unlike PV, few commercial-scale solar-thermal plants are currently in operation, and accordingly much less is known about their economics (Desai & Bandyopadhyay, 2017). The first utility-scale solar-power seawater desalination plant, Saudi Arabia’s Al Khafji, relies on 119 hectares of PV modules to produce 45.7MW of power for the plant’s expected 60,000 cubic meters of water per day capacity. However, its planned completion in early 2017 has been delayed (Tarantola, 2017).
Existing estimates suggest that for a medium-scale desalination plant with a daily production capacity in the 5,000 cubic meter per day range, either PV or solar-thermal technology could be applied at a cost of approximately US$2 per cubic meter, roughly double the cost of conventionally-powered seawater RO desalination (Fiorenza, Sharma, & Braccio, 2007). Where fuel costs are high, the cost differential may narrow. The cost of diesel-powered seawater RO desalination was estimated at US$1.18-1.56 for a small-scale plant in a remote coastal area of Egypt, for example, compared with US$1.21 using PV (Souman, Sorour, & Abulnour, 2015).
A major source of uncertainty in these cost estimates is that of energy and water storage. As in the case of wind, solar-powered desalination plants would have to overcome the problem of intermittency either by relying on batteries or pumping and storing water during periods of high insolation. Both options add considerably to capital costs, but advances in battery storage could help improve economics, as could using multiple power sources (Napoli & Rioux, 2015). One estimate suggests that combining solar and wind power with battery storage could result in desalination water production costs as low as US$0.6 per cubic meter by 2030, for example, making it competitive with conventional RO technology costs (Caldera, Bogdanov, & Breyer, 2016).
Public Policy Challenges
Solar-powered desalination offers the promise of simultaneously addressing two of humanity’s greatest sustainable development challenges, water scarcity and climate change. However, there are currently no clear commercial pathways for the development of large-scale solar-powered desalination. In the short term, small-scale niche applications may offer the greatest potential to expand the use of solar-powered desalination.
Simple direct desalination using solar stills or PV-based desalination is already highly attractive for remote, off-grid areas because of the high costs of transporting water or connecting to the electricity grid. Novel nano-scale technologies, including advanced ceramics, promise to increase the efficiency of these processes to the point where it appears likely that most small communities can eventually be supplied with affordable water using direct or PV-powered desalination (Finnerty, Zhang, Sedlak, Nelson, & Mi, 2017); (Dongare, et al., 2017).
Solar desalination plants also hold substantial promise to increase water availability in water-scarce inland areas with brackish water deposits. Such deposits are found in many irrigated agricultural regions, where drainage water is usually too saline to reuse directly, and in many energy production areas, where it is often associated with oil and gas-bearing geological formations. Desalination using any technology is more economical for brackish water because salt concentrations are far lower (Chaouchi, Zrelli, & Gabsi, 2007). Depending on the salinity of the source water, the energy requirements of brackish water desalination can be less than 1 kWh per cubic meter, as compared to 3 to 7 for seawater, and recovery rates can be as high as 90%, compared with about 60% for seawater (National Research Council, 2008).
For larger-scale coastal desalination plants, however, it seems certain that continued investment in research, development, and deployment is needed from both companies and governments. Indeed, desalination has always relied heavily on public investment. The U.S. government invested some $1.5 billion in desalination research during the second half of the twentieth century, which led to development of the first commercial use of RO technologies (National Research Council, 2008).
Enthusiasm for at least some government research support appears to be strong. Masdar, a company owned by the government of the United Arab Emirates, has invested in a small-scale, 1,500 cubic meter per day solar desalination pilot project that aims to reduce energy use by 40% compared with existing conventional desalination plants (Harrington, 2015). In September of 2017, the U.S. Department of Energy committed US$15 million to fund up to ten solar desalination demonstration projects (U.S. Department of Energy, 2017). Nonetheless, such efforts must be continued and expanded if utility-scale solar desalination is ever to become a reality.
But while these investments are necessary, policy reforms to improve the economics of sustainable desalination are arguably even more important to encourage its expansion. At present, desalination is generally only cost effective if water tariffs are raised to finance its high-capital and operation costs, or on extensive government subsidies. Yet while on the one hand subsidies are unsustainable for most governments, on the other hand the political pressure to keep water prices low is considerable, and rates must typically be approved by a government regulator.
One solution to these challenges is to ensure that water prices reflect the true cost of obtaining water from different sources. While desalination is extremely expensive, the full cost of exploiting surface and groundwater resources, especially for ecosystem services, is considerable, and accounting for these externalities could improve the cost-effectiveness of desalination. China, for example, has reformed water prices in several areas to feature differential pricing for ground and surface water to better account for these externalities. When coupled with carbon pricing or taxation policies, these water reforms could better support the expanded use of solar-powered desalination (National Research Council, 2008).
Informed by proper accounting of externalities, solar-powered desalination can be an important solution for some water-stressed urban and rural areas. Yet whatever the source of energy, desalination will always involve difficult trade-offs. The optimal sites for desalination plants are often either in high-land-value waterfront areas or in environmentally sensitive coastal zones, often requiring a protracted permitting and environmental impact assessment process. For these reasons, desalination is best employed as a strategy of last resort, when all reasonable water conservation and efficiency measures have been exhausted (Zetland, 2016). Nonetheless, as the U.S. National Academy of Science has pointed out, the value of water supply assurance is such that many utilities and municipalities will nonetheless be willing to pay the price of desalination to ensure adequate supplies of clean water in times of drought (National Research Council, 2008). It is essential that when they do so, they take steps to ensure desalination contributes to an energy- as well as water-sustainable future.
Scott Moore
Director of China Programs and Strategic InitiativesScott Moore is a faculty fellow at the Kleinman Center for Energy Policy and the Director of China Programs and Strategic Initiatives. He is also a practice professor of political science.
Badiuzzaman, P., McLaughlin, E., & McCauley, D. (2017). Substituting freshwater: Can ocean desalination and water recycling capacities substitute for groundwater depletion in California? Journal of Environmental Mangement, 203, 123-135.
Caldera, U., Bogdanov, D., & Breyer, C. (2016, May 2). Local cost of seawater RO desalination based on solar PV and wind energy: a global estimate. Desalination, 385, 207-216.
Carta, J., Gonzalez, J., & Subiela, V. (2003). Operational analysis of an innovative wind powered reverse osmosis system installed in the Canary Islands. Solar Energy, 75(2), 153-168.
Chaouchi, B., Zrelli, A., & Gabsi, S. (2007, November 5). Desalination of brackish water by means of a parabolic solar concentrator. Desalination, 217(1-3), 118-126.
Desai, N., & Bandyopadhyay, S. (2017, January). Line-focusing concentrating solar collector-based power plants: a review. Clean Technologies and Environmental Policy, 19(1), 9-35.
Dongare, P., Alabastri, A., Pedersen, S., Zodrow, K., Hogan, N., Neumann, O., . . . Halas, N. (2017). Nanophotonics-enabled solar membrane distillation for off-grid water purification. Proceedings of the National Academy of Sciences, 114(27), 6936-6941.
Finnerty, C., Zhang, L., Sedlak, D., Nelson, K., & Mi, B. (2017). Synthetic graphene oxide leaf for solar desalination with Zero Liquid Discharge. Environmental Science and Technology, 51(20), 11701-11709. Retrieved from Environmental Science and Technology.
Fiorenza, G., Sharma, V., & Braccio, G. (2007). Techno-Economic Evaluation of a Solar-Powered Water Desalination Plant. In L. Rizzuti, Solar Desalination for the 21st Century (pp. 33-41). Springer.
Harrington, K. (2015, December 11). Masdar launches solar desalination pilot program. Retrieved December 4, 2017, from American Institute of Chemical Engineers: https://www.aiche.org/chenected/2015/12/masdar-launches-solar-desalination-pilot-program
International Atomic Energy Agency. (2000). Introduction of Nuclear Desalination: A Guidebook. Vienna: International Atomic Energy Agency.
Kajenthira, A., Siddiqi, A., & Anadon, L. D. (2012). A new case for promoting wastewater reuse in Saudi Arabia: Bringing energy into the water equation. Journal of Environmental Management, 102, 184.
Loutatidou, S., Liosis, N., Pohl, R., Ouarda, T., & Arafat, H. (2017, April 15). Wind-powered desalination for strategic water storage: Techno-economic assessment of concept. Desalination, 408, 36-51.
Maleki, A., Pourfayaz, F., & Ahmadi, M. (2016, December 1). Design of a cost-effective wind/photovoltaic/hydrogen energy system for supplying a desalination unit by a heuristic approach. Solar Energy, 139, 666.
MENA Report. (2016, August 4). United Arab Emirates: Tapping the Earth’s energy for zero-carbon seawater desalination. MENA Report.
Napoli, C., & Rioux, B. (2015). A Framework for Comparing the Viability of Different Desalination Approaches. Riyadh: King Abdullah Petroleum Studies and Research Center.
National Research Council. (2008). Desalination: A National Perspective. Washington, D.C.: National Academies Press.
Poseidon Water. (2010). Desalination Worldwide. Retrieved December 3, 2017, from Seawater Desalination Huntington Beach Facility: http://hbfreshwater.com/desalination-101/desalination-worldwide
Reif, J., & Alhalabi, W. (2015). Solar-thermal powered desalination: Its significant challenges and potential. Renewable and Sustainable Energy Review, 48, 152-165.
Semiat, R. (2008). Energy issues in desalination processes. Environmental Science and Technology, 42(22), 8193.
Souman, E., Sorour, M., & Abulnour, A. (2015). Economics of Renewable Energy for Water Desalination in Developing Countries. International Journal of Economics and Management Sciences, 5(305), 1-5.
Steel, W. (2017). Look to Windward: the case for wind powered desalination. Retrieved December 4, 2017, from Water and Wastewater International: http://www.waterworld.com/articles/wwi/print/volume-31/issue-6/featured-articles/look-to-windward-the-case-for-wind-powered-desalination.html
Stieglitz, R., & Docksai, R. (2009, Nov/Dec). Why the world may turn to nuclear power. The Futurist, 43(6), 16-22.
Stokes, J., & Horvath, A. (2009). Energy and Air Emission Effects of Water Supply. Environmental Science & Technology, 43(8), 2680.
Talbot, D. (2015, March/April). Megascale Desalination. MIT Technology Review. Retrieved from https://www.technologyreview.com/s/534996/megascale-desalination/
Tarantola, A. (2017, October 27). Seawater desalination will quench the thirst of a parched planet. Retrieved December 4, 2017, from Engadget: https://www.engadget.com/2017/10/27/seawater-desalination-quench-parched-planet/
U.N. Food and Agriculture Organization. (2016). AQUASTAT. Retrieved December 3, 2017, from Food and Agriculture Organization of the United Nations: http://www.fao.org/nr/water/aquastat/main/index.stm
U.S. Department of Energy. (2017, September 27). Energy Development Launches Up to $15 Million to Tackle Solar Desalination. Retrieved December 4, 2017, from Office of Energy Efficiency and Renewable Energy: https://energy.gov/eere/articles/energy-department-launches-15-million-tackle-solar-desalination
Voutchkov, N. (2016, August 17). Desalination—Past, Present and Future. Retrieved December 3, 2017, from International Water Association: http://www.iwa-network.org/desalination-past-present-future/
Zetland, D. (2016). Society, Politics and Desalination. In D. Zetland, A Multidisciplinary Introduction to Desalination (pp. 559-574). Aalborg, Denmark: River Publishers.
Ziolkowska, J., & Reyes, R. (2016). Impact of socio-economic growth on desalination in the US. Journal of Environmental Management, 167, 15-22.