Rare Earth Elements: A Resource Constraint of the Energy Transition
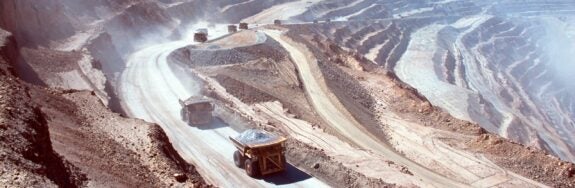
Electric motors and turbines are an integral part of decarbonization. While these technologies may not directly produce carbon emissions, many of them require specific rare earth elements. Extracting these elements is an energy-intensive and heavily polluting process, complicating the transition to "clean" energy.
At a Glance
Policy Insight
Other dimensions of energy sustainability must also be considered as we transition away from fossil fuels.
Introduction
Climate change is presenting humans with an unprecedented challenge: the need to wean ourselves off of a group of valuable natural resources; not because of scarcity or cost, but because of their long-term global pollution impacts. Although the combined capabilities of wind, solar, hydropower, and geothermal technologies have the potential to harness near limitless amounts of energy from our environment, they are not free from the limitations of resource availability.
On the contrary, the clean energy transition will require economic mobilization on a scale not seen since the industrial revolution, and will strain the global production of silicon, cobalt, lithium, manganese, and a host of other critical elements (Behr 2019).
One group of natural resources that may prove essential for the next generation of electric motors and turbines are the rare earth elements (REEs)—17 elements consisting of scandium, yttrium, and the 15 lanthanides (Institute of Rare Earths and Strategic Metals, n.d.).
We note that elements such as lithium and cobalt are frequently referred to as “rare earth metals” due to their relative scarcity, but do not belong to this chemical classification and are not the subject of this digest (Motavalli 2010). The long-term sustainability of lithium and cobalt mining is itself a significant economic and political challenge of the energy transition, but is beyond the scope of this research.
Despite their global importance, the production or REEs has become increasingly concentrated in China over recent years. Not only does this present a geopolitical and economic risk to most of the developed world, but it is also indicative of possible future supply constraints which could interrupt progress toward a decarbonized future.
In this digest, we explore how and why this consolidation of the REE market occurred, and what the tradeoffs are of increased global production. We also discuss several policies to ensure that future production of REEs does not slow the adoption of clean energy technology.
What Are Rare Earth Elements?
Historically, REEs have primarily been utilized in industrial processes for their catalytic properties. They are essential to the petrochemical industry in breaking down large molecules into smaller hydrocarbons suitable for use in fuels (Akah 2017). Recently, the demand for REEs has dramatically increased due to the permanent magneticity that is exhibited by their alloys (See Figure 1).
Alloys of neodymium (Nd) and samarium (Sm) are strong magnets that can withstand relatively high temperatures, making them ideal for a wide variety of electronics and defense applications. The strongest known magnet is an alloy of neodymium with iron (Fe) and boron (B), abbreviated NdFeB. Other REEs such as dysprosium (Dy) and praseodymium (Pr) can be added to NdFeB to change the performance properties of these magnets (Constantinides 2012).
The clean energy technologies sector is expected to increase demand for REEs considerably, because strong magnets are critical in the construction of direct drive wind turbines and permanent magnet motors for electric vehicles (DOE 2019; Hanejko 2020).

Direct drive wind turbines currently make up a small fraction of the total wind energy capacity in the United States. However, their high efficiency and relatively low maintenance requirements compared to other turbine technologies have put direct drive wind turbines in consideration for large-scale offshore wind installations.
Similarly, motors containing REE permanent magnets have recently become the standard choice for electric vehicles due to their high efficiencies. With the growing need to forgo fossil fuels, the demand for REEs from the wind and electric vehicle industries is projected to increase in the near future (Alonso et al., 2012).
What impact this might have on price is open to debate and will be further explored throughout this digest. Over the last decade, for example, despite increasing demand, prices have remained relatively stable because of cheap Chinese production (Bloomberg 2021).
The Industry Then and Now
Rare earth elements are widely distributed throughout the earth’s crust, but generally in low concentrations. The concentration of individual REEs depends on the type of mineral deposit being mined, and REEs are often found alongside radioactive elements such as thorium and uranium (Rhodes 2011). Production is thus geographically limited to certain REE-rich mineral deposits and often requires the separation and safe disposal of dangerous waste material.
The mining of rare earth elements for industrial use is a relatively modern undertaking. The first REE mine was Mountain Pass, located in California’s Mojave Desert (Green 2019). The mine opened in 1952 to supply uranium for military and research purposes, and methods for isolating and removing rare earth elements were developed to secure a pure supply of uranium. Today, similar methods are used to remove uranium and thorium during REE production.
It was not until the early 1960s with the widespread adoption of color television that a commercial use for rare earth elements was finally found (Mills 2019). Europium, a REE found in large quantities at Mountain Pass, was essential for producing the red phosphors used in early color television sets (Encyclopedia Britannica, n.d.). Since then, the number of applications for rare earth elements has continued to grow, and today several REEs (including dysprosium, neodymium, terbium, europium, and yttrium) are considered critical materials worldwide (Bauer et al., 2011).
From the 1960s to the 1990s, Mountain Pass remained the only large-scale source of rare earth elements globally (See Figure 2). However, around the turn of the millennium, policy makers and manufacturers determined that it no longer made sense to pay for REEs mined in accordance with the relatively strict environmental protection laws in the United States (Hudson et al., 1999).
By 2002, Mountain Pass mine was defunct, and by 2010 China had cornered 95% of global REE production by providing the cheapest material (King, n.d.). The development of this sector in China subsequently revealed the serious environmental costs of large-scale REE production and significantly altered the global supply chain (Ives 2013).
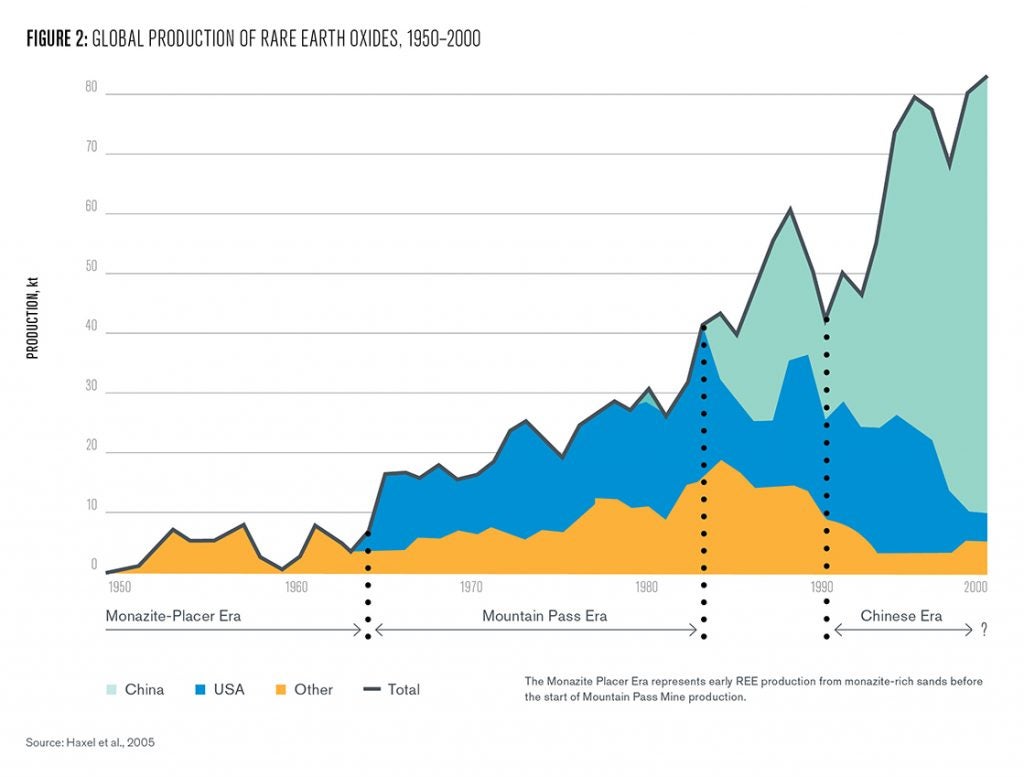
Over the past decade, nations have been reckoning with the outsized power that China has on the global supply chain of REEs. Following a Chinese embargo on sales of REEs to Japan in 2010, countries around the world were finally alerted to the economic risks inherent in having a foreign power control the supply and distribution of these critical materials (Bradsher and Wong 2010).
There have since been calls for the World Trade Organization to prevent China from limiting REE exports. Meanwhile, China has begun to crack down on illegal mining within its borders and is rethinking its willingness to accept pollution and ecosystem destruction in exchange for economic gain (Standaert 2019).
Even with new trade partnerships emerging, and modest growth in REE production in the U.S., Australia, and Brazil, the future of the international REE market remains highly uncertain (Vella 2020; Boyd 2020). Environmental risks, regulatory costs, and low-density deposits still prevent most countries from competing directly with China.
If China’s commitment to a cleaner and healthier environment persists, the world could see a dramatic increase in REE prices. On the other hand, if China does not institute lasting regulations on the REE mining industry, and other countries relax existing environmental controls and permitting restrictions in order to increase competition, global production could increase, and prices could drop. These environmental risks may be difficult to avoid since a secure supply of REEs will be essential to a fully decarbonized energy system.
Understanding Future Demand
The history and present state of the REE supply chain exhibits the important role these materials already play in the world economy. Projections of a sharp increase in demand over the coming decades raise several questions about the future environmental impacts and supply risks to this industry. A 2012 MIT study by Alonso et al. thoroughly explores this question of future supply, and projects total global demand out to 2035 under five divergent scenarios.
One of these scenarios uses the IEA Blue Map scenario to estimate future wind and automotive electrification (IEA 2010). This model only seeks to reduce global carbon emissions by 50% by 2050. Given our understanding of climate sensitivities in 2021, these projections should be considered far too limited to reach global emissions targets. They provide us with a conservative estimate of demand for the purpose of this analysis (IPCC 2018).
Under this scenario the study projects that by 2035 global demand for REEs will reach close to 450,000 tons per year, compared to approximately 200,000 tons per year today (USGS 2021). This represents more than a doubling in the size of the industry in just 15 years, which is again overly conservative according to present day decarbonization targets.
Furthermore, the rate of demand growth in Alonso et al. accelerates rapidly, as do projections of wind turbine and EV production out to 2050, indicating that this increase in industry demand is only the beginning of a pattern of accelerating growth that will likely last for decades (Larson et al., 2020).
As technology advances and demand for clean energy solutions intensifies, overall production of REEs will have to scale to accommodate growing demand for only a small handful of elements needed for magnets—specifically neodymium (Nd) and dysprosium (Dy). Whereas Alonso et al. predicts that 2035 demand for yttrium (Y) and terbium (Tb) will only be approximately 250% of 2010 supply, 2035 demand for Dy will be over 2500% the supply of Dy in 2010.
REEs are typically co-located in small concentrations, so global mine operations may need to produce a significant excess of many lesser-used elements to produce sufficient Dy. This effort to match production of elements to their relative demand is called mine yield balancing and promises to be a growing challenge in the REE industry. Industrial use of REEs is a relatively recent economic development and uses for these elements developed to accommodate their natural abundance and take advantage of low market prices.
It is, therefore, uncertain how the global market will respond to the excess supply and lower prices of REEs not used for magnet production, since uses for many other REEs are still limited. Because production of these minerals is almost always complementary, market demand for each element is important to consider in investment and operational decision-making.
If insufficient demand for these other elements emerges, it could significantly increase the long-term cost of critical elements such as neodymium and dysprosium. For emerging and sustainable energy solutions to effectively utilize rare earth elements, a higher premium for these materials will likely be necessary.
Environmental Costs
Regional ecosystems can be significantly altered by the presence of mines, both physically and chemically. Site preparation, access roads, and ancillary facilities lead to direct—and often absolute— destruction of the proximate environment, while pollution from mine processes and storage of residual tailings can lead to widespread chemical imbalances and toxic contamination (Filho 2016; Xiang 2016; Ganguli 2018).
REE mine tailings contain processing chemicals, salts, and radioactive materials. Tailings are particularly problematic in REE mining, because of the significant waste-to-yield ration. (Filho 2016; Xiang 2016). For every ton of REEs that are produced, there are 2,000 tons of mine tailings, including 1 to 1.4 tons of radioactive waste (Filho 2016).
Tailings are most commonly stored in isolated impoundment areas called tailing ponds. These ponds require complex management, especially if the tailings contain high concentrations of uranium or thorium. Poor construction or catastrophic failure can lead to long-term and widespread environmental damage and contamination of surface or groundwater (Filho 2016).
Other significant sources of pollution include aerosols and fugitive dust from tailing impoundments, which are created from cutting, drilling, and blasting rock. This pollution can accumulate in surrounding areas (Filho 2016), causing respiratory issues and also contaminating food sources—as plants absorb the airborne pollutants.
An example of this is the tailing pond for the Bayan Obo mine in China. Villages in the surrounding area have experienced elevated rates of both cancer and respiratory illness, indicating that the tailings are not being properly stored (Xiang 2016). While many of these issues exist for other types of mining, they are particularly problematic for REEs, because of the large volume and radioactivity of tailings.
Policy Solutions
As we look to the future of clean energy and the role of rare earth elements in decarbonization, we are faced with a difficult tradeoff. REEs such as dysprosium and neodymium offer an effective way to improve the efficiency and performance of crucial clean energy technologies. However, the production of these elements is environmentally and economically costly.
Global prices have remained relatively affordable in recent years only because of China’s lax environmental regulations and illegal mining operations. But China is now reckoning with the true costs of toxic, acidic, and radioactive run-off and leaching.
If demand for REEs continues to grow as expected, the pressure on nations to increase domestic production at the expense of the environment will increase. For example, there is growing interest in expanding U.S. production of REEs beyond Mountain Pass (Garcia and Smith 2020).
Failure to avoid an increase in environmental impacts from larger-scale production would jeopardize the sustainability of renewable energy technologies utilizing REEs and could partially offset their emissions benefits. This would in many ways undermine the goals of decarbonization and would further stress ecosystems and communities that have already been affected by climate change.
In order to assure that the transition to carbon-free energy minimizes environmental costs and promotes global sustainability, policy support for clean energy solutions should be coupled with streamlined environmental regulation and sustainability incentives. Fundamentally, all human development carries environmental impacts.
In the case of rare earth elements, policies intended to encourage the production and sale of wind turbines and electric vehicles should be designed in a way that encourages continued innovation in sustainable motor designs and REE recycling. These policies should also be coupled with environmental regulations and incentives to ensure REEs are secured in a sustainable and relatively benign way.
This policy plan is subtly distinct from a policy that might be designed with the sole intention of encouraging the rapid adoption of EV’s and wind turbines. Such a policy would simply incentivize producers to employ the best and most efficient technologies—in this case, REE magnet motors and generators. It would then be up to the market to signal an increase in production of REEs to meet this demand, and an inevitable tension would arise between resource supply, affordability, and environmental sustainability.
By coupling technology adoption incentives with demand management policies and environmental regulations, policymakers can optimize the speed and sustainability of the transition to clean energy by addressing these competing environmental priorities upfront.
In addition to tax incentives, permit streamlining, and carbon pricing for wind energy and electric vehicles, a sustainable suite of REE policies would include increased environmental regulations for mining. These regulations would in turn control the supply of REEs and consequently promote innovation in magnets using underutilized REEs or smaller quantities of REEs altogether.
Managing Demand
While companies including Siemens Gamesa and Tesla are making a strong case for the added efficiency and performance of permanent magnets, minor efficiency and performance losses may be an acceptable trade-off for avoiding stiff competition over a globally limited resource (Siemens Gamesa, n.d.; Desai 2018). The case of rare earth elements suggests that the newest or objectively “best” technological solution may not always be the most practical or sustainable solution.
Because environmental externalities are, for the most part, not priced appropriately, technologies are typically only assessed according to their production cost and performance. By thinking ahead to the resource demands of the energy transition, one can recognize the value and importance of simple technologies with little dependence on scarce or difficult to secure resources, even if that sustainability comes with trade-offs to price or performance.
One pathway to demand reduction is to increase the efficiency of REE use within existing magnet designs. Through innovations in material composition and the production processes, some manufacturers have been able to synthesize magnets with similar strengths to current REE magnets, but with much lower concentrations of REEs (Toyota 2018). Permanent magnets can also be made entirely without REEs, but these designs typically exhibit additional drawbacks (Widmer 2015).
Another option is to further develop and encourage the use of motors and generator technologies that do not utilize permanent magnets. Gearbox wind turbines featuring induction generators use almost no REEs. The main drawback of this design is the need for mechanical gearboxes to speed up the relatively slow rotations of the turbine rotor. These gearboxes wear out over time and require regular maintenance. Electric vehicle motors can also be made using induction designs that require little or no REEs, albeit with lower efficiencies.
Despite these drawbacks, generators and motors based on electromagnetic induction have been in use for decades and are still generally cheaper than technologies utilizing REEs (Widmer 2015). The current wind capacity in the U.S. is predominantly supported by induction generators and most of the earlier Tesla models (Roadster, Model S, and Model X) run on induction motors. Further technological developments in these areas or using hybrid designs that incorporate small amounts of REEs to increase efficiencies can help manage the overall demand of REEs.
A final method for reducing demand for REEs in wind power and EV markets would be to reuse and recycle existing rare earth magnets. Unfortunately, the infrastructure and systems needed to achieve REE reprocessing are severely underdeveloped. REE reuse and recycling is limited by inefficient and costly collection, global shipping constraints, low REE concentration in most products, and long lifetimes of products (Filho 2016). Figure 3 demonstrates the lifecycle of REEs, including existing recycling methods.
***fig 3
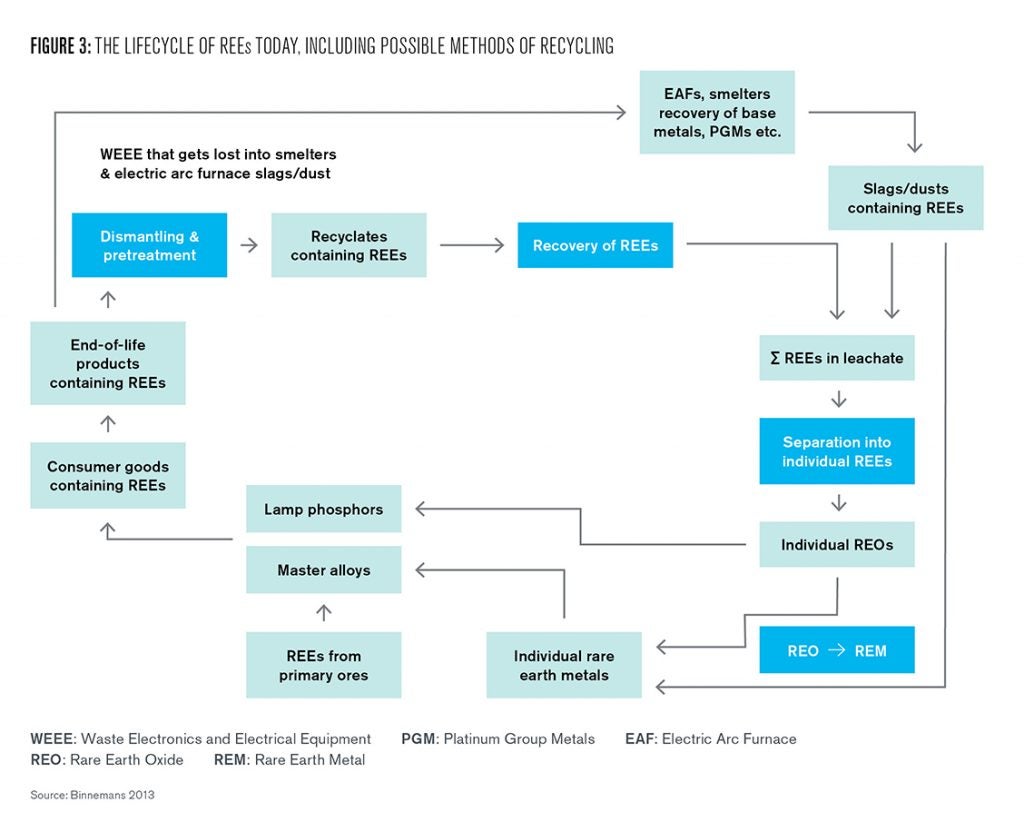
Retired wind turbines and EV motors are a source of recyclable REEs, since their magnets are relatively large and can be recovered without too much difficulty. In some cases, large magnets can simply be reused and do not need to undergo the recycling process (Binnemans 2013). However, clean energy applications of REEs face another roadblock to scalable recycling: insufficient stock material.
Recycled REEs can only ever make up a portion of demand equivalent to the total stock of REEs in currently available retired equipment. Recycling in industries such as wind power and electric vehicles, where demand is increasing exponentially and manufactured equipment is designed for a decade of use or more, are inherently limited in this way. Therefore, it will be decades before the stock of retiring magnets is sufficient to meet a considerable share of new demand.
Managing Impacts
One of the most powerful and efficient tools for regulating environmentally risky and large-scale industry operations is the application of a pollution pricing scheme, or tax. In recent policy discussions, this policy tool is commonly discussed in the context of carbon emissions, but a similar scheme could be applied to mine tailing and wastewater from REE mining operations.
Efficient and effective policies should tax mine waste according to the environmental and community vulnerability and value within the potentially impacted region. Imposing strict pollution fees for key contaminants of mining operations could create a market incentive for resource producers to take additional steps to limit their pollution and provide a source of funding for federal or regional regulatory efforts. Having geography-specific pricing also incentivizes mining to take place in areas of lower environmental and community risk.
There are several innovative methods mine operators can use to reduce their environmental and community impacts. One possibility is to utilize reserves of unconventional deposits of REE. This includes deep ocean deposits, or waste streams from slag or iron ore tailings (Filho 2016; Xiang 2016; Cook 2016; Peelman 2018).
In some studies, iron ore tailings have been found to have relatively high REE concentrations (Peelman 2018). Coal and coal ash, which can contain 68 to 404 ppm rare earth elements, has a similar REE content to clays that are already mined in China (Cook 2016).
However, there are still a number of environmental concerns with all of these options, and processes for the extraction of REEs from these sources are still nascent (Filho 2016). It is not yet clear if the costs of these methods will make them worthwhile for REE producers to pursue.
Other possibilities for reducing the environmental impact of REE mining involve improving the technology and processes being used in existing mining efforts, including finding ways to maximize recovery of REEs from mined material, upgrade recycling routes of e-waste and tailings to decrease waste disposal, and improve tailing treatment and pond or dam safety (such as better monitoring, infrastructure, etc.) (Xiang 2016).
Lastly, there has been some work examining the possibility of recovering thorium from REE waste. This could not only eliminate some of the radioactive waste from REE mining but could also offset costs if a reliable market for thorium emerges as part of the energy transition. This is a possible technical solution that deserves further exploration by researchers in chemistry and chemical engineering.
Akah, Aaron. 2017. “Application of Rare Earths in Fluid Catalytic Cracking—A Review.” Journal of Rare Earths 35, no. 10. Accessed April 6, 2021, https://www.sciencedirect.com/science/article/pii/S1002072117609980.
Alonso, Elisa, A. Sherman, T. Wallington, M. Everson, F. Field, R. Roth, and R. Kirchain. 2012. “Evaluating Rare Earth Element Availability: A Case with Revolutionary Demand from Clean Technologies.” Environmental Science and Technology 46, no. 6. Accessed April 6, 2021, https://pubs.acs.org/doi/pdf/10.1021/es203518d.
Bauer, Diana, D. Diamond, J. Li, M. McKittrick, D. Sandalow, and P. Telleen. 2011. “Critical Material Strategy.” U.S. Department of Energy. Accessed April 6, 2021, https://www.energy.gov/sites/prod/files/DOE_CMS2011_FINAL_Full.pdf.
Behr, Peter. 2019. “100% Renewable Grid Could Cost Trillions— Study” E&E News. Accessed April 6, 2021, https://www.eenews.net/energywire/2019/07/01/stories/1060678667.
Binnemans, Koen, P.Jones, B. Blanpain, T. Gerven, Y. Yang, A. Walton, and M. Buchert. 2013. “Recycling of Rare Earths: A Critical Review.” Journal of Cleaner Production. Accessed April 7, 2021, https://solvomet.eu/wp-content/uploads/2017/05/JCLEPRO_Binnemans_REE_Recycling_May2013.pdf.
Boyd, John. 2020. “U.S. and Japan Seeking to Break China’s Grip on Rare Earths Production.” IEEE Spectrum. Accessed April 6, 2021, https://spectrum.ieee.org/tech-talk/semiconductors/materials/us-and-japan-seeking-to-break-chinas-grip-on-rare-earths.
Bradsher, Keith and Edward Wong. 2010. “China’s Ban on Selling Rare Earth Minerals to Japan Continues.” The New York Times. Accessed April 6, 2021, https://www.nytimes.com/2010/10/11/business/global/11rare.html.
Constantinides, Steve. 2012. “The Demand for Rare Earth Materials in Permanent Magnets.” Arnold Magnetic Technologies Corporation. Accessed April 6, 2021, https://www.arnoldmagnetics.com/wp-content/uploads/2017/10/Demand-for-rare-earth-materials-in-permanent-magnets-Constantinides-COM-2012-psn-hi-res.pdf.
Desai, Pratima. 2018. “Tesla’s Electric Motor Shift to Spur Demand for Rare Earth Neodymium.” Reuters. Accessed April 7, 2021, https://www.reuters.com/article/us-metals-autos-neodymium-analysis/teslas-electric-motor-shift-to-spur-demand-for-rare-earth-neodymium-idUSKCN1GO28I.
Editorial Board. 2021. “How to Ease China’s Hold on Rare Earths” Bloomberg Opinion. Accessed April 6, 2021, https://www.bloomberg.com/opinion/articles/2021-03-25/how-u-s-should-ease-china-s-rare-earth-dominance.
Encyclopedia Britannica. n.d. “Phosphor.” Accessed April 6, 2021, https://www.britannica.com/science/phosphor.
Filho, Walter. 2016. “Chapter 17: An Analysis of the Environmental Impacts of the Exploitation of Rare Earth Materials.” Rare Earths Industry. Elsevier Inc. Accessed April 7, 2021, https://reader.elsevier.com/reader/sd/pii/B9780128023280000176?token=A3FA7CD540E2219F8565ABE4F8FFB625CBBFC6CD4B335DCF85C020F4A6A6C37DE18DFC410761193E3B12A33F37FCEFE0&originRegion=us-east-1&originCreation=20210407161907.
Ganguli, Ranjive and Douglas R. Cook. 2018. “Rare Earths: A Review of the Landscape.” MRS Energy & Sustainability. Accessed April 7, 2021, https://www.cambridge.org/core/services/aop-cambridge-core/content/view/4F699CE5A563C492E7E654FDA4D0D78E/S2329222918000077a.pdf/div-class-title-rare-earths-a-review-of-the-landscape-div.pdf.
Garcia, Cardiff and Stacey Vanek Smith. 2020. The Strategic Value of Rare Earths.” National Public Radio. Accessed April 6, 2021, https://www.npr.org/transcripts/949590270.
Green Jeffery. 2019. “The Collapse of American Rare Earth Mining—and Lessons Learned.” Defense News. Accessed April 6, 2021, https://www.defensenews.com/opinion/commentary/2019/11/12/the-collapse-of-american-rare-earth-mining-and-lessons-learned/.
Hanejko, Fran. 2020. “Induction vs. Permanent Magnet Motor Efficiency: Auto Electrification.” Horizon Technologies. Accessed April 6, 2021,https://www.horizontechnology.biz/blog/induction-vs-permanent-magnet-motor-efficiency-auto-electrification.
Haxel, Gordon, J. Hedrick, and G. Orris. 2005. “Rare Earth Elements—Critical Resources for High Technology.” U.S. Geological Survey. Accessed April 6, 2021,https://pubs.usgs.gov/fs/2002/fs087-02/.
Hudson, T., F. Fox, and G. Plumlee. 1999. “Metal Mining and the Environment.” American Geosciences Institute. Accessed April 6, 2021, https://www.americangeosciences.org/critical-issues/faq/what-are-regulations-mining-activities.
International Energy Agency. 2010. Energy Technology Perspectives 2010. IEA. Accessed April 6, 2021, https://www.iea.org/reports/energy-technology-perspectives-2010.
IPCC. 2018. Summary for Policymakers—Special Report: Global Warming of 1.5°C. IPCC. Accessed April 6, 2021, https://www.ipcc.ch/sr15/chapter/spm/.
Institute for Rare Earths and Strategic Metals. “Rare Earths/Rare Earth Elements/REE.” Accessed April 6, 2021, https://en.institut-seltene-erden.de/rare-earths-and-metals/rare-earth/.
Ives, Mike. 2013. “Boom in Mining Rare Earths Poses Mounting Toxic Risks.” Yale Environment 360. Accessed April 6, 2021, https://e360.yale.edu/features/boom_in_mining_rare_earths_poses_mounting_toxic_risks.
Jowitt, Simon, T. Werner, Z. Weng, and G. Mudd. 2018. “Recycling of the Rare Earth Elements.” Green and Sustainable Chemistry. Accessed April 7, 2021, https://www.researchgate.net/publication/323502917_Recycling_of_the_Rare_Earth_Elements.
King, Hobart. n.d. “REE – Rare Earth Elements and their Uses.” Geology.com. Accessed April 6, 2021, https://geology.com/articles/rare-earth-elements/.
Larson E. Larson, C. Greig, J. Jenkins, E. Mayfield, A. Pascale, C. Zhang, J. Drossman, R. Williams, S. Pacala, R. Socolow, EJ Baik, R. Birdsey, R. Duke, R. Jones, B. Haley, E. Leslie, K. Paustian, and A. Swan. 2020. “Net-Zero America: Potential Pathways, Infrastructure, and Impacts, interim report” Princeton University. Accessed April 6, 2021, https://netzeroamerica.princeton.edu/img/Princeton_NZA_Interim_Report_15_Dec_2020_FINAL.pdf.
Mills, Rick. 2019. “How the U.S. Lost the Plot on Rare Earths.” Mining.com. Accessed April 6, 2021, https://www.mining.com/web/us-lost-plot-rare-earths/.
Motivalli, Jim. 2010. “Forget Lithium—It’s Rare Earth Minerals That Are in Short Supply for EV’s.” CBS News. Accessed April 6, 2021, https://www.cbsnews.com/news/forget-lithium-its-rare-earth-minerals-that-are-in-short-supply-for-evs/.
Natural Resources Canada. 2021. “Rare Earth Elements.” Government of Canada. Accessed April 6, 2021, https://www.nrcan.gc.ca/our-natural-resources/minerals-mining/minerals-metals-facts/rare-earth-elements-facts/20522.
Peelman, S., D. Kooijman, J. Sietsman, and Y. Yang. 2018. “Hydrometallurgical Recovery of Rare Earth Elements from Mine Tailings and WEEE.” Journal of Sustainable Metallurgy. Accessed April 7, 2021, https://link.springer.com/content/pdf/10.1007/s40831-018-0178-0.pdf.
Rhodes, Chris. 2011. “Rare Earth Elements and Thorium Power.” Forbes. Accessed April 6, 2021, https://www.forbes.com/sites/energysource/2011/03/28/rare-earth-elements-and-thorium-power/?sh=3d60a6952d76.
Siemens Gamesa. n.d. “SG 8.0-167 DD Offshore Wind Turbine.” Accessed April 7, 2021, https://www.siemensgamesa.com/en-int/products-and-services/offshore/wind-turbine-sg-8-0-167-dd.
Standaert, Michael. 2019. “China Wrestles with the Toxic Aftermath of Rare Earth Mining.” Yale Environment 360. Accessed April 6, 2021, https://e360.yale.edu/features/china-wrestles-with-the-toxic-aftermath-of-rare-earth-mining.
Toyota. 2018. “Toyota Develops New Magnet for Electric Motors Aiming to Reduce Use of Critical Rare-Earth Element by up to 50%.” Accessed April 7, 2021, https://global.toyota/en/newsroom/corporate/21139684.html.
U.S. Department of Energy. 2019. “Advanced Wind Turbine Drivetrain Trends and Opportunities.” Office of Energy Efficiency & Renewable Energy. Accessed April 6, 2021, https://www.energy.gov/eere/articles/advanced-wind-turbine-drivetrain-trends-and-opportunities.
U.S. Geological Survey. 2021. “Mineral Commodities Summaries 2021.” U.S. Geological Survey. Accessed May 6, 2021: https://pubs.usgs.gov/periodicals/mcs2021/mcs2021.pdf
Vella, Heidi. 2020. “Australia and the U.S.: A Rare, Rare-Earth Partnership.” Mining Technology. Accessed April 6, 2021, https://www.mining-technology.com/features/australia-and-the-us-a-rare-rare-earth-partnership/.
Xiang, Huang, G. Zhang, A. Pan, F. Chen, and C. Zheng. 2016. “Protecting the Environment and Public Health from Rare Earth Mining.” Earth’s Future. Accessed April 7, 2021, https://agupubs.onlinelibrary.wiley.com/doi/full/10.1002/2016EF000424.
Oscar Serpell
Deputy DirectorOscar Serpell oversees all student programming, alumni engagement, faculty and student grants, and visiting scholars. He is also a researcher, writer, and policy analyst working on research initiatives with students and Center partners.
Wan-Yi “Amy” Chu
Assistant Professor, Mills CollegeWan-Yi “Amy” Chu is an assistant professor in Chemistry at Mills College in Oakland CA and a former postdoctoral researcher for the Goldberg Group at the University of Pennsylvania.
Benjamin Paren
Alumni Research FellowBenjamin Paren is an alumni research fellow at the Kleinman Center and a postdoctoral research associate in the Research Laboratory of Electronics at the Massachusetts Institute of Technology.