Electricity Storage and Renewables: How Investments Change as Technology Improves
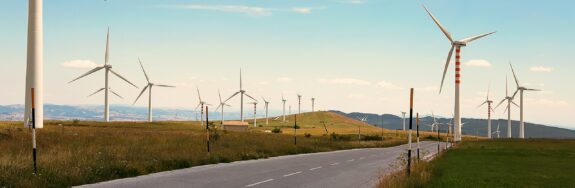
Wind has become the cheapest energy source, and energy storage is becoming cheaper every year. Together they could become the main source of electricity worldwide. When will this happen and which storage technology will prevail?
At a Glance
Key Challenge
To successfully transition to more sustainable electricity grids, we need to understand how multi-hour storage and renewables interact, when and how much to invest in them and how improving technology costs, different markets and carbon pricing affect these decisions.
Policy Insight
For large-scale, multi-hour energy storage, low-efficiency, low-cost technologies, e.g., thermal, will be profitable sooner than batteries. For these long-term load shifting storage requirements, the ratio of cost to efficiency decides which technology to invest into.
Introduction
Facing the realities of climate change, scarce natural resources, and geopolitical uncertainty, many consumers, utilities, and regulators move toward renewable energies. In 2019, of the 245 gigawatts (GW) of power generation capacity that were added around the world, 72% was renewable, of which wind and solar accounted for the lion’s share (IRENA 2019).
Yet, generation capacities are not created equal. While conventional power plants predictably produce energy for well over 90% of their scheduled time, wind turbines produce electricity at an erratic and unpredictable rate, and the sun does not always shine on a solar panel.
Simultaneously, customers expect electricity to be available at the flip of a switch and demand varies across days, weeks, and seasons. Hence, when shifting energy grids toward a more renewable future, one needs to match demand with an increasingly variable and less controllable supply. To ensure grid stability, we must rely on large-scale energy storage.
Yet, actual market adoption of storage is miniscule, and it is currently not well understood what technology or regulation is needed to drive capacity investments. We will explore what is required, from a strategic level, to see large-scale storage adoption.
4 Large-Scale Storage Trends
In recent years, there have been four concurrent developments that make a grid-level storage approach sought-after, technically feasible, and potentially profitable.
The first development is political in nature, with many national and regional governments enacting regulation that requires minimum renewable energy generation ratios in future decades. These include California, aiming at 100% renewables by 2045 (U.S. Senate 2015), Germany (Bundestag 2016) set to achieve 50% by 2030, and China, which has committed to 35% by the same year (Shen 2018).
The second trend is the ever-decreasing cost for fossil-free technologies, with wind generation costs down 40% and photovoltaic prices down by 70 to 80%, compared to 2009 (IRENA 2017). This has rendered renewables increasingly competitive. Refer to Figure 1 to see the historical cost development of both technologies (levelized cost of energy, or LCOE, refers to a plant’s average generation cost over its lifetime).
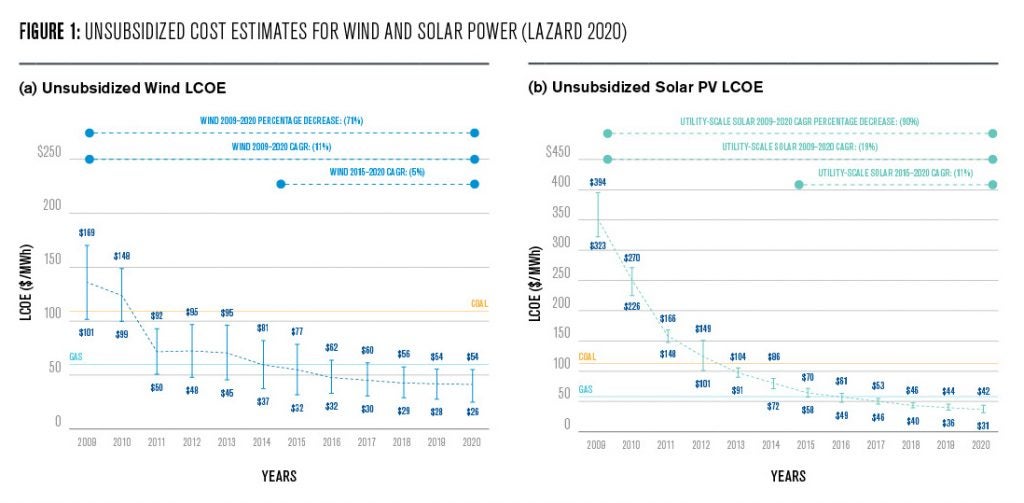
The third trend, which lends further credibility to the previous two, is that carbon emissions are under scrutiny in international treaties, such as the Paris and Katowice climate accords (EU Commission 2015), and further environmental regulations are investigated by economic scholars and (non)governmental institutions (World Bank 2017). Thus, renewables constitute a promising solution to replace existing carbon-intensive capacity, irrespective of eventual future emission enforcement mechanisms.
Lastly, the cost of energy storage has been decreasing steadily over the past several years, making industry-scale storage economically viable (e.g. lithium-ion cost decreased from $1,183 per kWh in 2010 to $137 per kWh in 2020). Tesla showcased in 2017 that multi-megawatt-hour (MWh) batteries can be built and operated profitably (Koziol 2017) and the industry’s exploitation of value stacking (using storage in multiple energy-related functions) improves their cost-benefit ratio.
Currently, several multi-100 MWh projects are under construction, some of which are designed to replace former power plants like the Moss Landing Power Plant in California. Consequently, the International Energy Agency predicts the global energy storage market to grow by 16% annually until 2030 (Cozzi and Gould 2018).
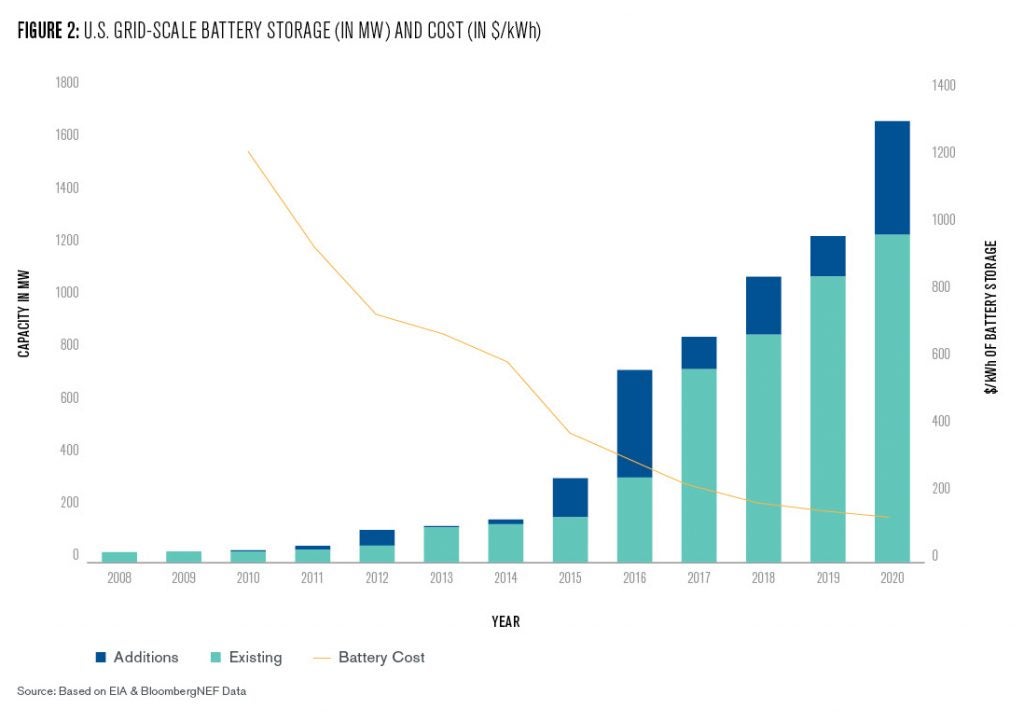
In combination, these trends make energy grids with high levels of renewable penetration (50 to 80%) politically desirable. Potentially, these grids offer both lower long-term costs and higher sustainability than conventional power grids.
Yet, while the direction of the energy transition seems clear, it is not understood what storage technology should be employed, let alone how much storage capacity should be built in any given market. In order to answer this question, we designed a model, which we calibrated with real-life market data from Germany, Texas, and the Northeastern United States to see 1) how much of today’s storage technologies one should optimally invest in, and 2) what policy makers and technology innovators can do in the coming years to change this outcome for the better.
(Gigawatt) Hours of Storage
Our model captures a dynamic that occurs in virtually every electricity market of the world—demand is higher during the day (when people are awake and industry is producing) than it is at night. Other, longer-term demand fluctuations—so called seasonality—exist as well, for example between weekdays and weekends or summer and winter. We focus on the daily fluctuations only as shorter-term storage becomes profitable before longer-term storage.
This fluctuating demand is currently met by adjusting gas, oil, or coal plant generation, so that the energy produced is equal to the energy demanded. However, soon, such demand could be served by relying on stored renewable energy. To be sure, renewables are stored already these days, but installed storage capacities (pumped hydro or batteries) are mainly used to respond to short demand spikes or to quickly regulate the grid frequency1 . This typically occurs on a time scale of seconds to minutes, while the kind of storage that we are investigating has an entirely different purpose and scale.
In our case, when renewables generate more energy than demanded, for example at night, the excess generation is stored in a large-scale storage unit and is then discharged whenever renewables generate less energy than demanded—for example during the day, when there is typically more demand than photovoltaic- and wind-power can supply. In our model, fossil fuels are only used if neither the current renewable generation nor the stored energy is sufficient to meet demand.
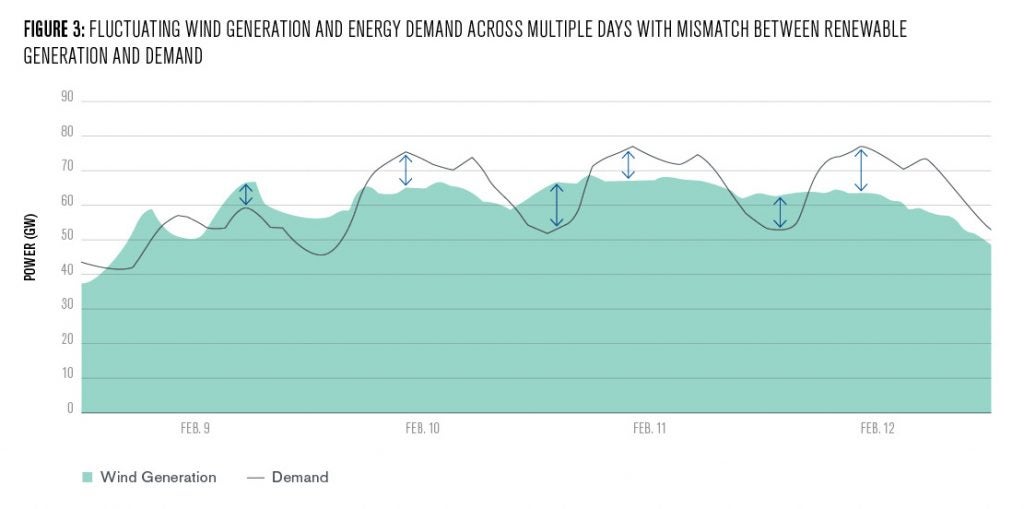
In other words, demand is met with renewables if possible, then with stored energy if possible, and then with fossil fuels as a last resort. This is not only more sustainable, but also cheaper, because producing energy with renewables is almost costless.
Of course, one must invest to build a wind-turbine or a solar panel and pay for maintenance, but once this is done, the wind and sun generate power for (almost) free. Likewise, the storage capacity must be bought and installed upfront, but then is utilized with inexpensive renewable electricity. This is different from a conventional, fossil-fuel plant that may already exist but still needs costly gas or oil to burn for every unit of energy it produces.
Batteries or Thermal Technology
Regarding storage technologies, we differentiate alternatives on two key dimensions: cost and efficiency. Cost is simply how much it costs to purchase a certain capacity of storage (expressed in MWh), or how much power over how much time the storage unit can hold. Efficiency refers to how much energy is lost in each charging/discharging cycle.
This efficiency is a core metric for storage technologies, since a perfect system would not lose any energy in the charging/discharging process and return 100% of the originally stored energy. But in real-world situations, among other things, secondary reactions in a battery and mechanical losses in thermal systems lead to energy dissipation. Ideally, a very cheap and very efficient storage system would be preferred, but among current technologies there is a trade-off between the two dimensions.
Often, the first thing that comes to mind when considering storage is a battery. With high efficiency and wide use-cases from cellphones to cars to industrial storage, batteries are one of technologies with the largest capacity installations in recent years. Despite the falling cost of lithium-ion batteries (an average of 8% annually) (Lazard 2018), the major downside of this class of storage is still the high unit cost and the resource-intensive production process. Such batteries are an example of a high cost/high efficiency technology.
A competitor to this is thermal energy storage—systems in which energy is stored as heat in various conductive materials ranging from sand over concrete or salt to oils. Typically, these storage solutions have lower levels of efficiency than batteries but are also less expensive to build. To make the technologies comparable, we adjust the cost for the expected lifetime of the technology, given that batteries need to be replaced more frequently (approximately twice as often) as thermal storage.
Modelling Renewables, Storage, and Fossil-Fuel
Combining these pieces, we consider a utility that fulfills future demands through a combination of renewables, storage, and fossil fuels. The renewable technology in our model is wind because of its low cost and more stable generation over time compared to solar2 .
The technology can either be thermal or battery storage, as introduced above. The utility chooses to build 1) a certain capacity of wind power (i.e. a certain size of a wind park) and 2) a certain storage capacity.
With wind and storage in place, the utility then fulfills demand across 30 years. Demand is fixed, known in advance, and alternates between day and night (high and low) each 24-hour period. As said before, the wind-electricity is used first whenever possible—as it is the cheapest power source.
The actual wind-generation at each point in time is random and ranges from 0 to the maximum installed capacity, to capture the fluctuation in renewable generation. Any wind generation in excess of demand is stored in the storage unit, given that there is remaining capacity. Energy is discharged when needed.
Only if these two sources are not sufficient is the fossil fuel back-up plant used. The plant already exists, but the generated energy costs money for every unit used. This can be conceived of as a gas plant that is quickly able to respond to demand, but requires payment for fuel. The goal then for the firm or utility is to choose the optimal capacities at the beginning, so that across the 30 years, the combined payments for wind capacity, storage capacity, and back-up fossil fuel costs are as low as possible. We use contemporary cost-data for all technologies and markets (see Tables 1-3). For a detailed introduction of the model, please refer to the working paper (Kaps et al. 2021).
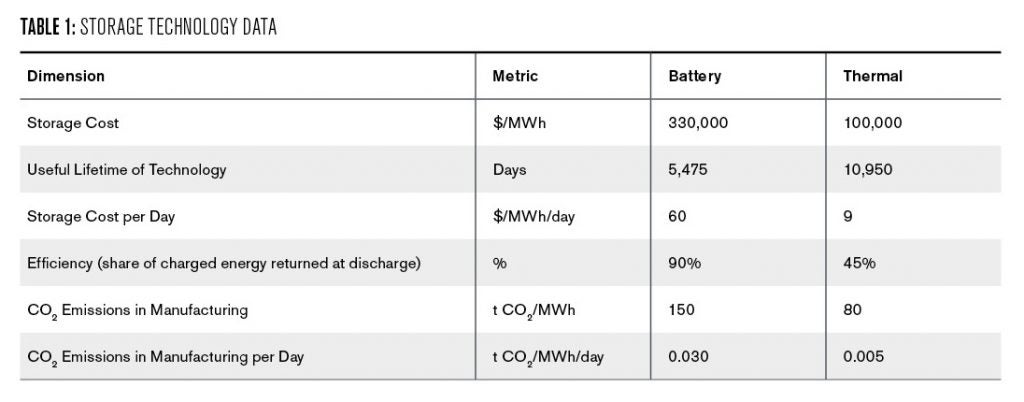
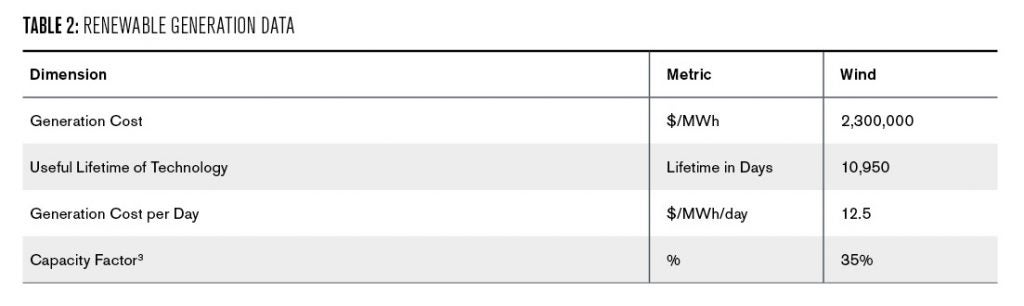
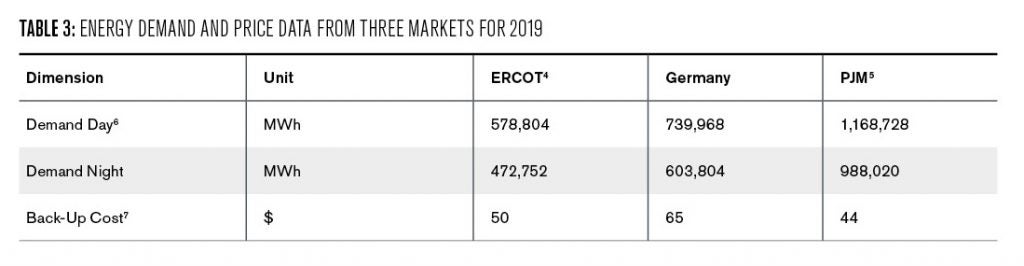
Obviously, this set-up is a stylized representation of reality and abstracts from certain aspects of real-life renewables and storage. However, it allows one to analytically dissect the dynamics of the capacity decisions and technology requirements. This level of generalizable insight cannot be achieved through pure simulation, which we also used to verify the model quality. Especially when fine-tuned with the real-life parameters, this approach lets one answer the high-level questions around when large-scale storage will become a reality. In the following, we present these real-world quantified results.
Renewables and Storage Capacity Are Not Always Complements
Many people believe that as more storage is built, more generation will be built, and vice versa, since investment of one type increases the value of an investment in the other type. However, we find that this is not always the case. At low-to-medium levels of renewable generation and storage, investing in one makes one invest into more of the other. With more renewables, there are more chances of excess generation and thus more opportunity to use storage profitably. And likewise, with more storage in place, more renewable capacity makes sense as excess generation is not lost but can be stored and used later.
However, at high levels of either capacity, investing into one capacity will reduce the profitability of the other. While this might seem unintuitive at first, imagine what would happen if one decided to increase wind-power to infinity: there would (nearly) always be more energy generated than demanded, removing any need for storage. Eventually, the dominant model could be one with a lot of renewable generation and very little storage (if wind becomes much cheaper than storage), or one in which generation is barely higher than expected demand, and a large storage capacity takes care of using any excess supply to meet any future unmet demand (if storage becomes much cheaper than generation). This challenges the popular notion that subsidizing either technology will automatically spur investment in the other.
Thermal Trumps Batteries for Large-Scale Storage
Despite the current investment focus on batteries, and contrary to academics (Kittner et al. 2017; Diouf and Pode 2015) and policymakers (Tsiropoulos 2018) who suggest that batteries may be the future technology of choice for storing electricity across multiple hours or days, we find that low-efficiency, cheap technologies such as thermal will be adopted sooner than high-efficiency, expensive ones such as lithium-ion batteries.
Once total storage capacity moves into the realm of hours of demand, when choosing between two storage technologies, the one with the lower cost-to-efficiency ratio will be invested into first. So, although thermal only has half the efficiency of a battery (45% vs 90%), it is currently five to six times cheaper, and is therefore the technology of choice. For a theoretical derivation of this ratio, please refer to the working paper (Kaps et al. 2021).
Storage Adoption Will Be Rapid
We show in Figure 3 what combination of cost and efficiency is required for a storage technology to be invested in. Although the exact values are calibrated to demands and prices of the German market, the structure, and dynamic holds true across all studied markets. It becomes evident that for a large range of cost–efficiency combinations, storage is not invested in at all—it simply isn’t profitable.
Yet, once the aforementioned cost-to-efficiency threshold is reached, any further improvement in technology or cost saving leads to rapidly increasing investment8 . This happens because at this threshold the renewable-plus-storage combination becomes cheaper than the fossil fuel back-up. This transforms the grid to one in which storage becomes the preferred way of matching fluctuating demand. It thus requires large-scale capacity investment to fulfill the role that was previously filled by things like gas plants. This rapid development is manifested, for example, in Texas, with about 0.6 GWh of storage currently installed but planning to add 7.2 GWh going forward (ERCOT 2019).
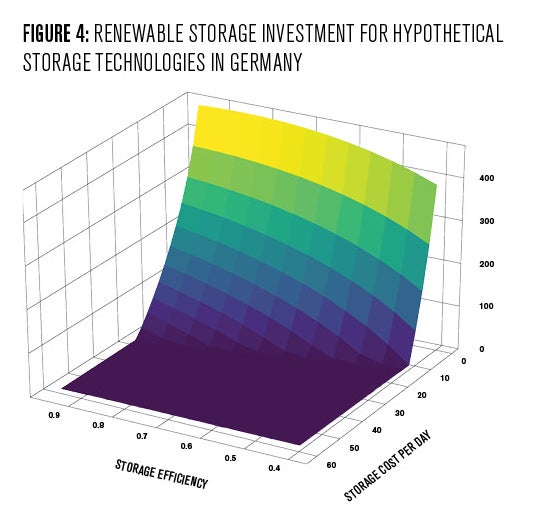
No One-Carbon-Price-Fits-All
In Table 3 we show what level of carbon price would be required in the three studied markets (Germany, Texas, and PJM in the Northeastern U.S.) to reach a) positive storage capacity, b) a storage capacity equal to 10% of total daily demand, and c) a storage capacity equal to 25% of daily demand.
While the carbon price is set independently of any storage, Table 3 shows that, depending on the specific market, the same relative storage capacities are reached at vastly different levels. With its high electricity prices, Germany needs no emission prices for a thermal storage capacity of up to 10% of daily demand, while the lowest back-up cost market, PJM, would need an $80 charge per ton.
Note that for batteries to become profitable, prices of more than $200 would be needed in almost all cases. These findings point to the fact that it is important for regulators to consider the implications of emission pricing or storage subsidies with respect to their idiosyncratic market situation, as these are by no means one-size-fits-all tools.
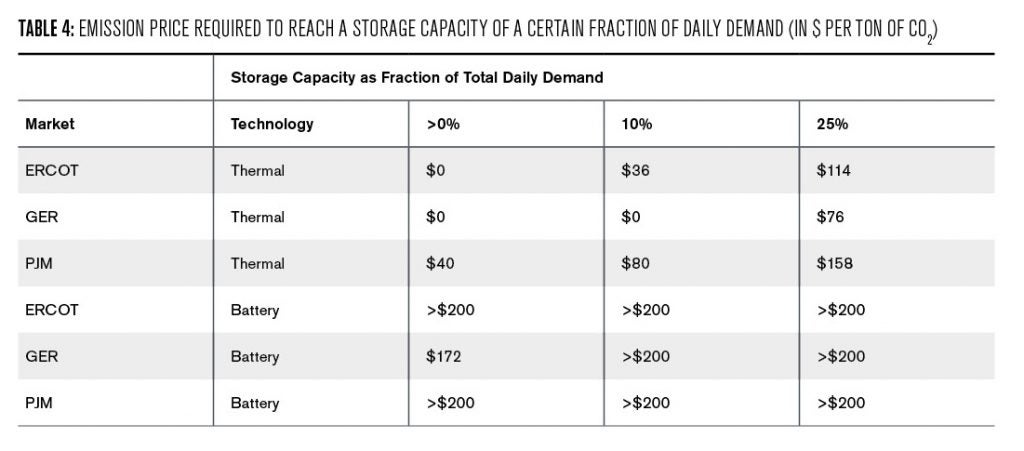
Also, it is noteworthy that while we predict thermal storage in Germany of more than 10% of demand without any emission tax, the country uses its high level of grid interconnection to European countries to run high levels of renewables (which our model also predicts) with less physical storage by importing and exporting energy across national borders—a “geographical” storage solution.
Decentralization Leads to Underinvestment in Storage
If one compares the capacity decisions of a monopoly utility investing into both renewable energy and storage to the capacity decisions of two separate firms (one investing into each), the decentralized firms invest in less renewables and less storage than the monopoly. Depending on market and carbon tax levels, the storage capacity investment is 30 to 70% lower in the decentral scenario than in the monopoly scenario. This causes a reduction in overall profits of up to 6% in the decentral scenario.
This holds true regardless of electricity price that the company owning the renewable gets paid for a unit of electricity that charges the storage firm’s capacity. The behavior occurs because the decentral parties separately optimize their own profit and do not focus on the benefit of jointly replacing the costly conventional generation. Yet, in many real-life markets today, storage and renewable generation are complements, meaning that increasing the capacity of one makes the other more useful. In separately optimizing profits, the firms undervalue the positive effects they have on each other and therefore undervalue storage and its ability to use (marginally) free renewable generation. While decentral company decisions are usually good for consumers, we show that there may be scenarios where separating investment into generation and storage would reduce storage and profits, relative to the monopoly case.
Fossil Fuels Will Remain
In electricity markets with high prices, such as Germany, we find that building a lot of renewable generation and large storage capacity is already attainable with today’s technologies. However, for renewable plus storage to generate high levels of electricity (e.g. 70%) at today’s electricity prices in markets with currently low prices, such as PJM in the US, thermal storage and wind generation would both need to become approximately 30% cheaper relative to 2019 levels.
At current technology maturation rates, these cost levels would be achieved in four years. Even if batteries remain too expensive, one can reach such levels profitably by building more renewable generation or relying on thermal storage. 80% renewable generation is approximately a decade away in most markets, mostly because it requires large storage capacity to become cheap enough to operate.
Yet, achieving 90% or more of generation through renewables is multiple decades away in all studied markets. This pattern showcases the increasing difficulty of replacing the flexible, fossil back-up when approaching very high levels of renewables. Of course, our model only looks at day-to-day discharge, whereas renewable-first grids will incorporate multiple different storage use-cases and revenue streams. Nevertheless, while renewables plus large-scale storage will likely become a reality soon, pivoting energy grids fully away from fossil generation may take an order of magnitude longer.
Christian Kaps
Doctoral StudentChristian Kaps is an operations Ph.D. candidate at The Wharton School. He received his master of science in supply chain management from Rotterdam School of Management.
Simone Marinesi
Assistant Professor, OperationsSimone Marinesi is an assistant professor of operations at The Wharton School. He received his master of science and Ph.D. in technology and operations management from the Institut Européen d’Administration des Affaires (INSEAD).
Serguei Netessine
Senior Vice Dean of Innovation & Global Initiatives, WhartonSerguei Netessine is Senior Vice Dean of Innovation and Global Initiatives and Dhirubhai Ambani Professor of Innovation and Entrepreneurship in the Operations, Information and Decisions Department at The Wharton School.
Bundestag, D. 2016. Gesetz f ̈ur den Ausbau erneuerbarer Energien (erneuerbare-energien-gesetz–eeg2014). Technical report. Revised 6/29/2015. Available online at http://www. gesetze-im-internet.de/bundesrecht/eeg2014/gesamt.
Cozzi, L. and T. Gould. 2018. World Energy Outlook 2018. Technical report, International Energy Agency.
Diouf, B. and R. Pode. 2015. “Potential of Lithium-Ion Batteries in Renewable Energy.” Renewable Energy 76, 375-380.
EU Commission. 2015. The Paris Protocol: A Blueprint for Tackling Global Climate Change Beyond 2020. COM 2015. 81.
Elliott, D., M. Schwartz, S. Haymes, D. Heimiller, G. Scott, L. Flowers, … and B. Phelps. 2010. “80 and 100 Meter Wind Energy Resource Potential for the United States.” (Poster) (No. NREL/PO-550-48036). National Renewable Energy Lab. (NREL), Golden, CO (United States).
ERCOT. 2019. Overview of Battery Additions in Ercot (Past, Present and Future). Technical report, Energy Reliability Council of Texas.
IRENA. 2017. Electricity Storage and Renewables: Costs and Markets to 2030. Technical report, International Renewable Energy Agency.
IRENA. 2019. Renewable Capacity Statistics 2019. Technical report. International Renewable Energy Agency.
Kaps, C., S. Marinesi, and S. Netessine. 2021. When the Wind of Change Blows, Build Batteries? Optimum Renewable Generation and Energy Storage Investments.
Kittner, N., F. Lill, and D. M. Kammen. 2017. “Energy Storage Deployment and Innovation for the Clean Energy Transition.” Nature Energy 2 (9), 17125.
Koziol, M. 2017. “South Australia Announces Tesla as Back of World’s Largest Battery.” The Sydney Morning Herald.
Lazard. 2018. Levelized Cost of Storage Analysis 4.0. Technical report. Lazard.
Lazard. 2020. Levelized Cost of Energy Analysis 14.0. Technical report. Lazard.
Shen, F. 2018. “China Steps up Its Push into Clean Energy.” Bloomberg News.
Tsiropoulos, I., D. Tarvydas, N. Lebedeva. 2018. Li-Ion Batteries for Mobility and Stationary Storage Applications. Technical report. European Commission.
U.S. Senate 2015. Bill no. 350.
World Bank 2017. State and Trends of Carbon Pricing 2017.
- Power grids operate at a set frequency (e.g. 50 Hertz in Europe, 60 Hertz in the U.S.), which is used to balance electricity generation and demand. Excess demand/generation will decrease/increase the grid frequency which is used as a signal by the grid operators to adjust power plants or shed load. Charging/discharging storage is used to stabilize the frequency at its target for short periods [↩]
- For our model to remain solve-able we can only use one renewable type and selected wind – the same general approach would hold true for solar. Ultimately, a real-life grid will most likely see a combination of solar and wind. [↩]
- What % of the nameplate capacity (e.g. 1 MW) is produced, on average, each hour (1MW*0.35 = 0.35MW/h [↩]
- Energy Reliability Council of Texas [↩]
- North Eastern US’s regional transmission organization [↩]
- Total demand during the day-subperiod (12 hours) [↩]
- Cost to generate 1 MWh using the back-up technology [↩]
- This adoption hypothesis assumes that enough renewable power generation can be built in a market to cover all demand, which easily holds in practice. For example, in the US wind alone could produce a multiple of the electricity demand (Elliott et al. 2010) [↩]