
U.S. CCS Ladder for Industrial Decarbonization
CCS has long been scrutinized for enabling fossil fuels, but in some industrial sectors that produce CO2 as a byproduct, CCS may be one of the only options for decarbonization today. Here’s a look at where CCS should be prioritized as a decarbonization strategy now—and for some sectors tomorrow.
The role of carbon capture and storage (CCS) in the energy transition is often scrutinized for enabling continued fossil fuel use, but in many cases CCS can help decarbonize industrial sectors. The question is: which ones?
In this new diagram, a CCS ladder for the United States, we lay out the role of CCS in industrial processes like mineral, metal, and petroleum-based sectors. This ladder can help inform policymakers of the prioritization of decarbonization opportunities across industrial sectors, to ultimately champion policies that support CCS deployment in areas where it will have the greatest impact.
CCS is a unique decarbonization option for industrial applications, because of the CO2 emissions produced from both burning fossil fuels (combustion emissions) and splitting molecules (process emissions). Combustion emissions may be eliminated through fuel switching (adoption of hydrogen or sustainable biomass-based fuels) or electrification, but process emissions are harder to avoid. Eliminating these emissions without CCS will require novel chemical pathways or alternative technologies, which may take up to a decade or more to become commercially viable.
Using a similar methodology as E3G and Bellona and the Clean Hydrogen Ladder, we evaluate the relevance of CCS deployment alongside a suite of decarbonization technologies in the United States. We used five criteria to establish a ranking from which CCS is best applied and where other decarbonization tools may take the lead:
- CCS feasibility
- CO2 mitigation potential
- Alternative decarbonization technology readiness and availability
- Potential for CCS to enable fossil-fuel or emissions-intensive technology lock-in
- Geospatial dispersion
A temporal element was also included by applying the criteria to near-term and long-term timeframes, assessing how economy-wide decarbonization impacts the ranking and CCS viability (Figure 1).
The alternative decarbonization methods we consider include: electrification with renewable energy, fuel switching to hydrogen (H2) or sustainably-sourced biomass, and increased energy and material efficiency. All three of these methods are essential to achieve net-zero GHG emissions in the U.S. by mid-century.
The deployment of decarbonization strategies must be considered regionally, accounting for local resources. Clustered large emitters co-located with geologic CO2 storage may favor CCS, while facilities located in states with a low carbon grid intensity or available sustainable biomass may favor other decarbonization strategies (that may also include CCS in the case of biomass conversion). In addition, subnational policies that include 100% clean electricity or net-zero GHG goals will be strategic locations for siting industrial decarbonization projects that rely on low-carbon energy resources. These important factors should be considered as we move forward with CCS policies.
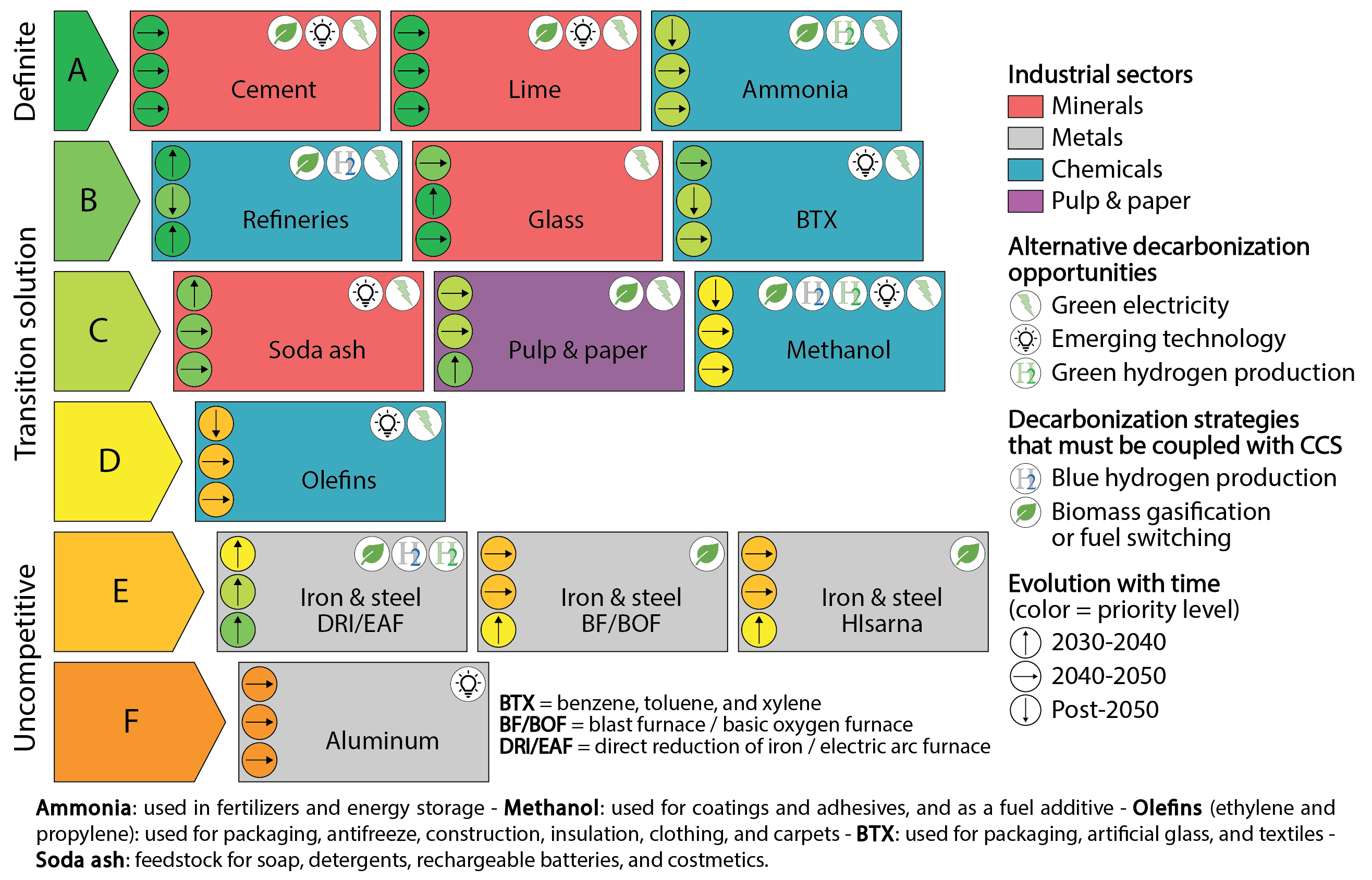
Key Takeaways
- Mineral-based industries (e.g., cement, lime, glass, and soda ash production) are favorable for CCS. They have substantial process emissions from a single source, so CCS may be a long-term viable solution for decarbonizing these sectors.
- Hydrogen production at refineries coupled to CCS is promising. It may displace the use of carbon-intensive fuel gas for distributed combustion emissions. Leveraging existing reforming technology at refineries can provide a bridge to refineries of the future, which may use clean hydrogen not just for heat, but, with CO2, as a chemical feedstock for producing synthetic fuels.
- CCS may be a transition solution for petrochemical industries as alternative technologies eliminate the need for CCS (such as emerging catalytic processes) reach higher technology readiness levels. Some approaches, such as synthesis gas (CO + H2) derived from advanced gasification of sustainable feedstocks (e.g., plastic, biomass, MSW), will still require CCS to achieve decarbonization and will likely be carried out in the long term in parallel to synthetic chemical pathways.
- CCS on reforming technology at refineries for H2 production and ammonia facilities today may include mostly fossil-sourced methane or natural gas but could transition to renewable methane/natural gas long term. This has the potential benefit of co-locating H2 production from renewable methane as a feedstock for ammonia synthesis for emissions mitigation associated with agriculture. This pathway would likely be deployed in parallel with approaches that include green hydrogen.
- CCS is a temporary solution for iron and steel production. As adoption of the direct reduction of iron steelmaking process grows, it may displace blast and basic oxygen furnaces for markets demanding higher quality steel (e.g., automotive industry). As steel recycling supply chains increase, the electric arc furnace may become increasingly adopted for markets demanding medium-to-lower quality steel products.
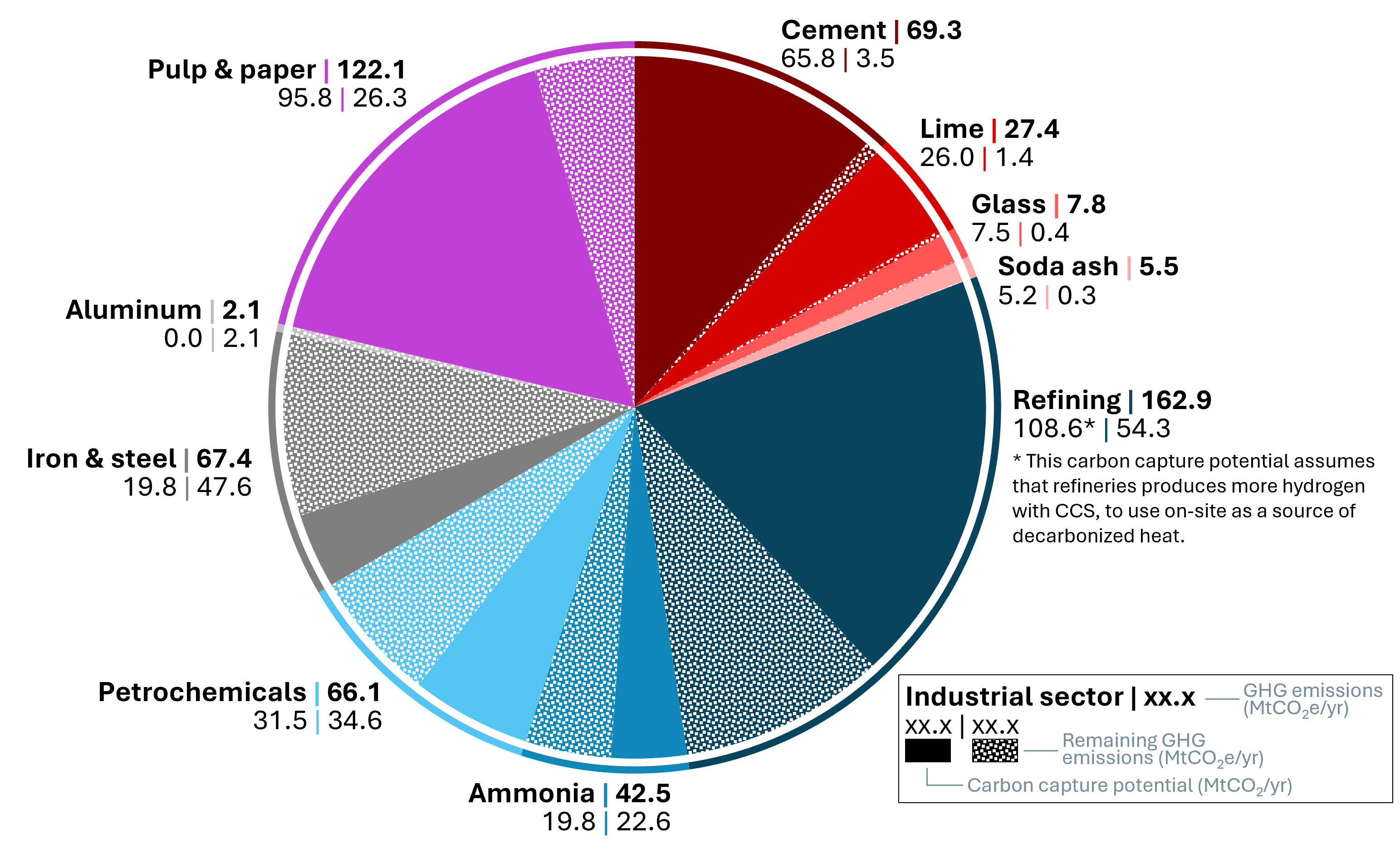
Overall, we see that nearly two thirds of industrial emissions could be decarbonized with CCS (Figure 2), with prioritization of industries that are high on the ladder (Figure 1). The remaining emissions have the potential to be decarbonized with alternative approaches (e.g., fuel switching, electrification, etc.), which will be dominating decarbonization strategies for the industries at the bottom of the ladder (Figure 1).
Maxwell Pisciotta
Postdoctoral Fellow, Clean Energy Conversions LabMax Pisciotta is a postdoctoral fellow in the Clean Energy Conversions Lab. They hold a PhD in Chemical Engineering from Penn whose research focused on carbon capture and carbon removal. They served on the Kleinman Center Student Advisory Board and completed the 2021 Kleinman Birol Fellowship.
Shelvey Swett
PhD Candidate , Chemical and Biomolecular EngineeringShelvey Swett is a third year PhD candidate in the Chemical and Biomolecular Engineering department at the University of Pennsylvania. Her work focuses on carbon capture and storage and on the recovery of critical minerals.
Hélène Pilorgé
Research Associate, Clean Energy Conversions LabHélène Pilorgé is a research associate with the University of Pennsylvania’s Clean Energy Conversions Laboratory. Her research focuses on carbon accounting of various carbon management solutions and on Geographic Information Systems (GIS) mapping for responsible deployment of carbon management.
Shrey Patel
PhD Candidate , Chemical and Biomolecular EngineeringShrey Patel is a second year PhD candidate in the Department of Chemical and Biomolecular Engineering at the University of Pennsylvania. His research focuses on the integration of low carbon energy sources with carbon capture and storage.
Jennifer Wilcox
Presidential Distinguished ProfessorJen Wilcox is Presidential Distinguished Professor of Chemical Engineering and Energy Policy. She previously served as Principal Deputy Assistant Secretary for the Office of Fossil Energy and Carbon Management at the Department of Energy.